Research Article | Open Access
Lipid Metabolism Pathway and Renal Tumor Therapy
Wenjun Wang1, Chunyan Xin2
1State Key Laboratory of Southwestern Chinese Medicine Resources, School of Ethnic Medicine, Chengdu University of Traditional Chinese Medicine, Chengdu, Si Chuan, 611137, P.R. China.
2Department of Pharmacy, Air Force Medical University, Xi 'an, Shaanxi, 710032, P.R. China.
Correspondence: Chunyan Xin (Department of Pharmacy, Air Force Medical University, 169 Changle West Road, Xi 'an, Shaanxi, 710032, P.R. China; Email: yxxjxb@fmmu.edu.cn).
Annals of Urologic Oncology 2023, 6(4): 13-18. https://doi.org/10.32948/auo.2023.12.30
Received: 16 Dec 2023 | Accepted: 30 Dec 2023 | Published online: 31 Dec 2023
Key words renal carcinoma, renal clear cell carcinoma, fatty acid metabolism, metabolic reprogramming, therapeutic targets
Clear cell renal cell carcinoma (ccRCC) is a subtype of the most common form of RCC. ccRCC gets its name because of the "clear" state of its cytoplasm rich in lipids and glycogen pathological staining. Studies have shown that fatty acids (FAs) play an important role in the membrane structure, energy metabolism and signal transduction of ccRCC, suggesting that the regulation of fatty acid levels plays an important role in the regulation of tumor development, including the regulation of fatty acid synthesis, modification, and uptake from the microenvironment and release of fatty acids from other lipid species [6, 7]. Numerous studies have confirmed that fatty acid metabolism plays an important role in the progression of ccRCC, and targeting fatty acid metabolism may be a potential way to reverse drug resistance and improve its prognosis.
This article summarizes the research progress of key factors of fatty acid metabolism in ccRCC and expounds their role in the progression of ccRCC, so as to find potential therapeutic targets of ccRCC and further clarify the potential clinical application prospect of interfering with fatty acid metabolism pathway in the treatment of kidney tumors.
Fatty acid transport protein (FATP) is a group of proteins involved in fatty acid uptake, which is mainly located in cells or cell membranes and plays a key role in long-chain fatty acid transport. Studies have shown that tumor cells can increase intracellular lipid content by enhancing the expression of FATPs to promote cancer progression and treatment resistance [10]. FATPs consists of six isomers, which are encoded by specific genes and are tissue-specific [11]. FATP2 is a common subtype found in kidney tissue and plays an important role in regulating exogenous fatty acid transfer and intracellular maintenance of lipid homeostasis [12]. FATP4 is a protein encoded by SLC27A4 gene [13]. Studies have found that FATP4 is highly expressed in renal tumor tissues and is associated with poor prognosis of RCC [8]. Tumor cells can overexpress fatty acid binding protein (FABP), which is involved in intracellular and intracellular lipid transport, to promote tumor progression. One study revealed that FABPl expression decreased in ccRCC tissues, while FABP5, FABP6 and FABP7 expression increased in ccRCC tissues compared to paracancer tissues [14]. Higher expression levels of FABP5, FABP6 and FABP7 and lower expression levels of FABPl are associated with poor prognosis of ccRCC [15].
ATP citrate lyase (ACLY) is a bridge between glucose metabolism and fatty acid synthesis and can catalyze the conversion of citric acid to acetyl-Coenzyme A(CoA), which directs excess glycolytic products to lipid synthesis, thus promoting tumor growth and differentiation. Studies have found that ACI and Y are highly expressed in ccRCC tissues, and the level of ACLY protein is positively correlated with the T stage and tumor grade of ccRCC [19].
Acetyl CoA carboxylase (ACC) is not only an enzyme that regulates fatty acid synthesis, but also participates in the oxidative metabolism of fatty acids [20]. ACC exists in cytoplasmic form (ACCA) and mitochondrial anchored form (ACCB), both of which catalyze acetyl CoA carboxylation to form malonyl CoA. ACCA is the main isomer mediating fatty acid synthesis, which can promote adipogenesis to meet the needs of cancer cells for rapid growth and proliferation. ACCB, an isoform near carnitine palmitoyl transferase 1 (CPT1), is a major regulator of CPT1 activity, and its expression and activity are inhibited in a variety of cancers [21].
Newly synthesized or over-consumed saturated fatty acids need to be saturated with stearyl CoA desaturase 1 (SCD1) in response to lipid toxicity and ER stress-induced apoptosis or iron death [21]. SCD1 is a regulatory enzyme that promotes lipid synthesis and plays an important role in cell membrane generation and signal transduction of cell metabolism. SCD1 is specifically highly expressed in ccRCC tumor tissues, and its expression level remains high throughout the progression of the disease [22].
Sterol regulatory element binding protein 1 (SREBP1) is a major regulator of fatty acid metabolism. SREBP1 is involved in the development of cancer and other diseases by regulating ACC, SCD1 and FASN transcription [23]. Yang et al. [24] found that lipid desaturation may be a metabolic marker of ccRCC, and demonstrated that SREBP1 is overexpressed in ccRCC cell lines and is necessary for lipid desaturation and cell growth in ccRCC. Zhang et al. [23] found that LINC01138 regulates the growth of ccRCC through SREBPI-mediated lipid desaturation and is associated with poor patient survival.
The expression and activity of CPT1 in ccRCC are decreased relative to normal kidneys, which is associated with poor prognosis of patients. Mechanistically, CPT1 is inhibited by HIF1 and HIF2, reducing fatty acid transport to mitochondria and forcing fatty acids into lipid droplets for storage [26]. It has been found that after specific knockout of peroxisome proliferator activated receptor Y(PPARγ) in hepatocellular carcinoma, the expression of protease involved in de novo synthesis of fatty acids is significantly reduced [24]. By staining with oil red O and BODIPY493/503, Sanchez et al. [27] found that PPARY was indispensable for the viability, proliferation and migration of ccRCC cells in vivo and in vitro, but the knockout of PPAR7 would not affect the expression of FASN and SCD1 in ccRCC cell lines. Nor did PPAR7 significantly affect the content of glyceryl ester. Therefore, PPAR7 did not affect the "transparent" phenotype of ccRCC.
Figure 1 shows that lipid metabolism pathway mechanism.
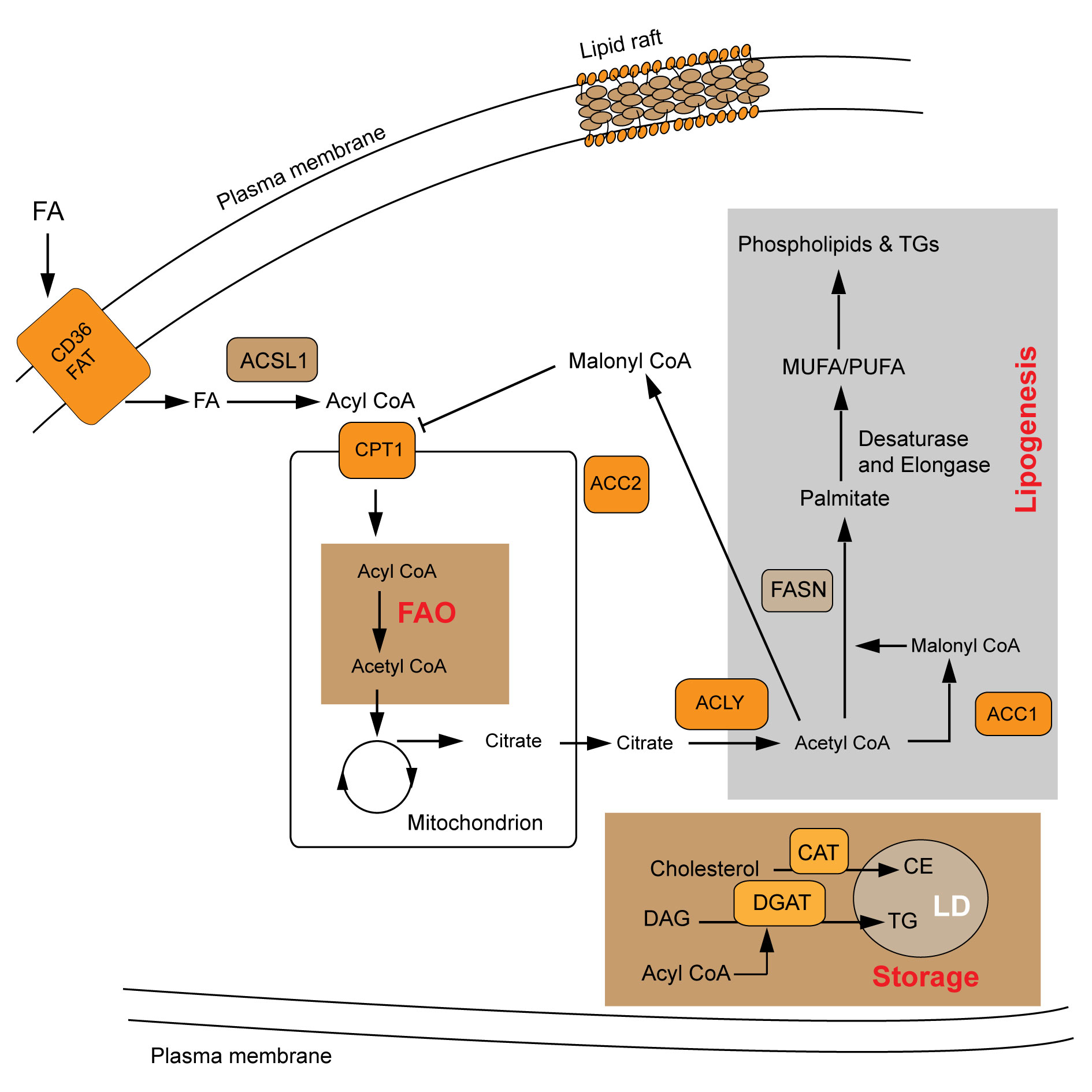
Fatty acids are first esterified into acyl CoA after entering the cell, and acyl CoA is transported to the mitochondria under the action of CPT1 to undergo fatty acid oxidation, which not only provides energy for tumor survival, but also provides reducing equivalent for tumor against oxidative stress. The uptake and metabolism of fatty acids are also regulated by the PPAR transcription factor family. Trastuzumab resistance has become a major obstacle in the treatment of HER-2 positive breast cancer patients. Studies have shown that AGAP2 ASl induced by mesenchymal stem cells can promote the stemness of breast cancer cells and induce trastuzumab resistance by upregulating CPT1 expression and inducing fatty acid oxidation [33]. Treatment of BRAF-mutated melanoma with MAPK inhibitors can result in significant tumor inhibition, but also lead to the prevalence of acquired drug resistance. Studies have found that PPARa-mediated CPTlA expression and increased fatty acid oxidation levels lead to MAPK inhibitor resistance in melanoma [34]. Thus, inhibitors targeting CPT1 or modulators of PPAR may be candidates for clinical treatment of cancer.
Citrate produced by tricarboxylic acid cycle is synthesized by ACLY, ACCs and FASN. Activated palmitate and other saturated fatty acids are desaturated by SCDs to produce monounsaturated fatty acids. Transcription of ACLY, ACCs, FASN and SCDs is also regulated by SREBP family [35]. ACLY and ACC are the rate-limiting enzymes of lipid synthesis, ACLY can divert excess glycolytic products to lipid synthesis. When selecting ACCl as a therapeutic target in breast cancer cells, attention should be paid to phosphorylation-mediated inactivation of ACCl, which can make breast cancer cells more aggressive and further induce tumor metastasis and recurrence by increasing the acetylation levels of intracellular acetylCoA and EMT-activating proteins [36]. In addition, some anti-obesity drugs are available, such as orlistat and other FASN inhibitors are also being tested in clinical trials in the hope of preventing tumor progression by inhibiting fatty acid synthesis [37, 38]. Orlistat has been shown to reduce angiogenesis and invasion in melanoma and to delay tumor growth in cisplatin-resistant ovarian cancer cells [39]. However, inhibition of FASN can compensatively cause CD36 up-regulation, and therefore, combined inhibition of FASN and CD36 may more favorably inhibit tumor progression [40]. SCD1 small molecule inhibitor A939572 can trigger the accumulation of saturated fatty acids, and in combination with tyrosine kinase or mTOR inhibitors can improve its efficiency and reduce its cytotoxicity [41]. Studies by Von Roemeling et al. [40] have shown that the combination of A939572 and tisirolimus can synergically inhibit the growth of ccRCC [22]. Wang et al. [42] found that SCD1 knockdown can inhibit the invasion of tumor cells, which may be caused by the reduced synthesis of fatty acids and the inhibition of Akt-mTOR signaling pathway. And subsequent studies found that interference with SCD1 combined with mTORCl/mTORC2 inhibitor AZDS055 in the treatment of ccRCC would produce more significant tumor inhibition effects. Kidney cancer cells, primary treated with A939572, proliferate more slowly than untreated cancer cells, and A939572 treated tumor cells had significantly higher mortality rates than untreated cancer cells after cisplatin treatment [43]. These results suggest that SCD1 inhibition significantly reduces cancer cell proliferation and increases cisplatin sensitivity, suggesting that this pathway may be related to ccRCC chemotherapy resistance. SREBPs is a major transcriptional regulator of adipogenesis. Its activation not only promotes the expression of lipid synthesis genes, but also promotes tumor progression and chemotherapy resistance. Therefore, in addition to being a prognostic marker, SREBPs can also be a potential therapeutic target for cancer treatment [44, 45].
Metabolic reprogramming is a hallmark of cancer and can play an important role in tumor progression by altering the metabolic capacity of tumor cells. Although most research on metabolic dysregulation in cancer has focused on carbohydrates, the importance of lipid metabolism-related alterations is beginning to be recognized. A large number of studies have confirmed that fat metabolism of tumor cells has an impact on biofilm synthesis, lipid synthesis and degradation, and signal transduction [48]. Abnormal fatty acid metabolism in RCC was first reported in 1987, and it was found by gas chromatography that cholesterol ester content in kidney tumor tissues was significantly higher than that in normal kidney tissues [49]. The reprogramming of fatty acid metabolism in RCC is mainly manifested in four aspects: (1) the role of de facto synthesis and exogenous uptake in cellular fatty acid pool; (2) the mechanism of molecular heterogeneity and carcinogenic signal transduction pathway regulating fatty acid metabolism; (3) Fatty acids as important mediators of cancer progression and metastasis reshape tumor microenvironment; therapeutic strategies targeting fatty acid metabolism in cancer [50].
In recent years, a variety of omics technologies, including proteomics, metabolomics and lipidomics, have made the research on ccRCC, a typical metabolic disease, enter a period of rapid development. Among them, the in-depth study on the mechanism of lipid metabolism in ccRCC is particularly eye-catching. As a metabolic disease characterized by classic molecular changes (VHL inactivation) and lipid deposition, ccRCC is an excellent model to study tumor lipid metabolism. Further study of the complex interaction between carcinogenic signaling pathways and dysregulation of fatty acid metabolism will provide broad prospects for revealing new metabolic pathways and improving targeted therapies.
None.
Ethical policy
All procedures performed in this study were in accordance with the ethical standards of the institutional and/or national research committee and with the 1964 Helsinki declaration and its later amendments or comparable ethical standards. Informed consent was obtained from all individual participants included in the study. Approval from institutional ethical committee was taken.
Availability of data and materials
All data generated or analysed during this study are included in this publication.
Author contributions
WJW: Conception, design of study and manuscript preparation, literature search and review; CYX: Manuscript writting, Approval for the final version of the manuscript and funding supports.
Competing interests
The authors have no competing interest.
Funding
None.
- Haineala B, Zgura A, Diaconu C, Mehedintu C, Bacinschi X, Anghel RM: Long-term Response After Stopping Immunotherapy in a Patient With Metastatic Renal Cancer. In Vivo 2021, 35(3): 1805-1810.
- Urbanski A, Paffenholz P, Schmidt T, Bruns CJ: Surgery for metastatic renal cell carcinoma. Onkologie 2023, 29(7): 613-621.
- Dell'Atti L, Bianchi N, Aguiari G: New Therapeutic Interventions for Kidney Carcinoma: Looking to the Future. Cancers 2022, 14(15).
- Karam JA, Zhang XY, Tamboli P, Margulis V, Wang H, Abel EJ, Culp SH, Wood CG: Development and Characterization of Clinically Relevant Tumor Models From Patients With Renal Cell Carcinoma. European Urology 2011, 59(4): 619-628.
- Linehan WM, Srinivasan R, Schmidt LS: The genetic basis of kidney cancer: a metabolic disease. Nature Reviews Urology 2010, 7(5): 277-285.
- Chen C, Zhao W, Lu XX, Ma YB, Zhang PZ, Wang ZC, Cui ZL, Xia QH: AUP1 regulates lipid metabolism and induces lipid accumulation to accelerate the progression of renal clear cell carcinoma. Cancer Science 2022, 113(8): 2600-2615.
- Zhang YJ, Wang H, Zhang J, Lv JW, Huang YR: Positive feedback loop and synergistic effects between hypoxia-inducible factor-2 and stearoylCoA desaturase-1 promote tumorigenesis in clear cell renal cell carcinoma. Cancer Science 2013, 104(4): 416-422.
- Kim YS, Jung J, Jeong H, Lee JH: High Membranous Expression of Fatty Acid Transport Protein 4 Is Associated with Tumorigenesis and Tumor Progression in Clear Cell Renal Cell Carcinoma. Dis Markers 2019, 2019: 5702026.
- Liao M, Li Y, Xiao A, Lu Q, Zeng H, Qin H, Zheng E, Luo X, Chen L, Ruan XZ et al: HIF-2α-induced upregulation of CD36 promotes the development of ccRCC. Exp Cell Res 2022, 421(2): 113389.
- Du W, Zhang L, Brett-Morris A, Aguila B, Kerner J, Hoppel CL, Puchowicz M, Serra D, Herrero L: HIF drives lipid deposition and cancer in ccRCC via repression of fatty acid metabolism. Nat Commun 2017, 8(1): 1769.
- Dourlen P, Sujkowski A, Wessells R, Mollereau B: Fatty acid transport proteins in disease: New insights from invertebrate models. Prog Lipid Res 2015, 60: 30-40.
- Acharya R, Shetty SS, Kumari NS: Fatty acid transport proteins (FATPs) in cancer. Chem Phys Lipids 2023, 250: 105269.
- Yen MC, Chou SK, Kan JY, Kuo PL: Solute Carrier Family 27 Member 4 (SLC27A4) Enhances Cell Growth, Migration, and Invasion in Breast Cancer Cells. Int J Mol Sci 2018, 19(11): 3434.
- Luis G, Godfroid A, Nishiumi S, Cimino J, Blacher S, Maquoi E, Wery C, Collignon A, Longuespée R, Montero-Ruiz L et al: Tumor resistance to ferroptosis driven by Stearoyl-CoA Desaturase-1 (SCD1) in cancer cells and Fatty Acid Biding Protein-4 (FABP4) in tumor microenvironment promote tumor recurrence. Redox Biol 2021, 43: 102006.
- Wu G, Zhang Z, Tang Q, Liu L, Liu W, Li Q, Wang Q: Study of FABP's interactome and detecting new molecular targets in clear cell renal cell carcinoma. J Cell Physiol 2020, 235(4): 3776-3789.
- Gong J, Shen S, Yang Y, Qin S, Huang L, Zhang H, Chen L, Chen Y, Li S, She S et al: Inhibition of FASN suppresses migration, invasion and growth in hepatoma carcinoma cells by deregulating the HIF-1α/IGFBP1 pathway. Int J Oncol 2017, 50(3): 883-892.
- Albiges L, Hakimi AA, Xie W, McKay RR, Simantov R, Lin X, Lee JL, Rini BI, Srinivas S, Bjarnason GA et al: Body Mass Index and Metastatic Renal Cell Carcinoma: Clinical and Biological Correlations. J Clin Oncol 2016, 34(30): 3655-3663.
- Xu W, Hu X, Anwaier A, Wang J, Liu W, Tian X, Zhu W, Ma C, Wan F, Shi G et al: Fatty Acid Synthase Correlates With Prognosis-Related Abdominal Adipose Distribution and Metabolic Disorders of Clear Cell Renal Cell Carcinoma. Front Mol Biosci 2020, 7: 610229.
- Granchi C: ATP citrate lyase (ACLY) inhibitors: An anti-cancer strategy at the crossroads of glucose and lipid metabolism. Eur J Med Chem 2018, 157: 1276-1291.
- Tamer F, Ulug E, Akyol A, Nergiz-Unal R: The potential efficacy of dietary fatty acids and fructose induced inflammation and oxidative stress on the insulin signaling and fat accumulation in mice. Food Chem Toxicol 2020, 135: 110914.
- Ali I, Khan A, Fa Z, Khan T, Wei DQ, Zheng J: Crystal structure of Acetyl-CoA carboxylase (AccB) from Streptomyces antibioticus and insights into the substrate-binding through in silico mutagenesis and biophysical investigations. Comput Biol Med 2022, 145: 105439.
- von Roemeling CA, Marlow LA, Wei JJ, Cooper SJ, Caulfield TR, Wu K, Tan WW, Tun HW, Copland JA: Stearoyl-CoA desaturase 1 is a novel molecular therapeutic target for clear cell renal cell carcinoma. Clin Cancer Res 2013, 19(9): 2368-2380.
- Sun Y, He W, Luo M, Zhou Y, Chang G, Ren W, Wu K, Li X, Shen J, Zhao X et al: SREBP1 regulates tumorigenesis and prognosis of pancreatic cancer through targeting lipid metabolism. Tumour Biol 2015, 36(6): 4133-4141.
- Lu X, Zhang X, Zhang Y, Zhang K, Zhan C, Shi X, Li Y, Zhao J, Bai Y, Wang Y et al: Metabolic profiling analysis upon acylcarnitines in tissues of hepatocellular carcinoma revealed the inhibited carnitine shuttle system caused by the downregulated carnitine palmitoyltransferase 2. Mol Carcinog 2019, 58(5): 749-759.
- Yang H, Deng Q, Ni T, Liu Y, Lu L, Dai H, Wang H, Yang W: Targeted Inhibition of LPL/FABP4/CPT1 fatty acid metabolic axis can effectively prevent the progression of nonalcoholic steatohepatitis to liver cancer. Int J Biol Sci 2021, 17(15): 4207-4222.
- Liu Y, Ma Z, Zhao C, Wang Y, Wu G, Xiao J, McClain CJ, Li X, Feng W: HIF-1α and HIF-2α are critically involved in hypoxia-induced lipid accumulation in hepatocytes through reducing PGC-1α-mediated fatty acid β-oxidation. Toxicol Lett 2014, 226(2): 117-123.
- Pramanik S, Sil AK: Cigarette smoke extract induces foam cell formation by impairing machinery involved in lipid droplet degradation. Pflugers Arch 2023, https://doi.org/10.1007/s00424-023-02870-4. Epub ahead of print.
- Zhang Q, Lin B, Chen H, Ye Y, Huang Y, Chen Z, Li J: Lipid metabolism-related gene expression in the immune microenvironment predicts prognostic outcomes in renal cell carcinoma. Front Immunol 2023, 14: 1324205.
- Chassen SS, Ferchaud-Roucher V, Gupta MB, Jansson T, Powell TL: Alterations in placental long chain polyunsaturated fatty acid metabolism in human intrauterine growth restriction. Clin Sci (Lond) 2018, 132(5): 595-607.
- Rachidi SM, Qin T, Sun S, Zheng WJ, Li Z: Molecular profiling of multiple human cancers defines an inflammatory cancer-associated molecular pattern and uncovers KPNA2 as a uniform poor prognostic cancer marker. PLoS One 2013, 8(3): e57911.
- Feng WW, Wilkins O, Bang S, Ung M, Li J, An J, Del Genio C, Canfield K, DiRenzo J, Wells W et al: CD36-Mediated Metabolic Rewiring of Breast Cancer Cells Promotes Resistance to HER2-Targeted Therapies. Cell Rep 2019, 29(11): 3405-3420.e3405.
- Guerrero-Rodríguez SL, Mata-Cruz C, Pérez-Tapia SM, Velasco-Velázquez MA: Role of CD36 in cancer progression, stemness, and targeting. Front Cell Dev Biol 2022, 10: 1079076.
- Han J, Qu H, Han M, Ding Y, Xie M, Hu J, Chen Y, Dong H: MSC-induced lncRNA AGAP2-AS1 promotes stemness and trastuzumab resistance through regulating CPT1 expression and fatty acid oxidation in breast cancer. Oncogene 2021, 40(4): 833-847.
- Campbell FM, Kozak R, Wagner A, Altarejos JY, Dyck JR, Belke DD, Severson DL, Kelly DP, Lopaschuk GD: A role for peroxisome proliferator-activated receptor alpha (PPARalpha ) in the control of cardiac malonyl-CoA levels: reduced fatty acid oxidation rates and increased glucose oxidation rates in the hearts of mice lacking PPARalpha are associated with higher concentrations of malonyl-CoA and reduced expression of malonyl-CoA decarboxylase. J Biol Chem 2002, 277(6): 4098-4103.
- Chi C, Harth L: Concomitant Inhibition of FASN and SREBP Provides a Promising Therapy for CTCL. Cancers (Basel) 2022, 14(18): 4491
- Xiong YX, Li N, Han MM, Ye F, Liu T, Ye HY, Zheng TT, Wu JJ, Li Y, Lv S et al: Rhodiola rosea polysaccharides-based nanoparticles loaded with DOX boosts chemo-immunotherapy for triple-negative breast cancer by re-educating Tumor-associated macrophages. Int J Biol Macromol 2023, 239: 124110.
- Ning Z, Guo X, Liu X: USP22 regulates lipidome accumulation by stabilizing PPARγ in hepatocellular carcinoma. Nat Commun 2022, 13(1): 2187.
- Schcolnik-Cabrera A, Chávez-Blanco A, Domínguez-Gómez G, Taja-Chayeb L, Morales-Barcenas R, Trejo-Becerril C, Perez-Cardenas E, Gonzalez-Fierro A, Dueñas-González A: Orlistat as a FASN inhibitor and multitargeted agent for cancer therapy. Expert Opin Investig Drugs 2018, 27(5): 475-489.
- Papaevangelou E, Almeida GS, Box C, deSouza NM, Chung YL: The effect of FASN inhibition on the growth and metabolism of a cisplatin-resistant ovarian carcinoma model. Int J Cancer 2018, 143(4): 992-1002.
- Gong J, Lin Y: Reprogramming of lipid metabolism in cancer-associated fibroblasts potentiates migration of colorectal cancer cells. Cell Death Dis 2020, 11(4): 267.
- Nashed M, Chisholm JW, Igal RA: Stearoyl-CoA desaturase activity modulates the activation of epidermal growth factor receptor in human lung cancer cells. Exp Biol Med (Maywood) 2012, 237(9): 1007-1017.
- Wang H, Zhang Y, Lu Y, Song J, Huang M, Zhang J, Huang Y: The role of stearoyl-coenzyme A desaturase 1 in clear cell renal cell carcinoma. Tumour Biol 2016, 37(1): 479-489.
- Lucarelli G, Ferro M, Loizzo D, Bianchi C, Terracciano D, Cantiello F, Bell LN, Battaglia S, Porta C, Gernone A et al: Integration of Lipidomics and Transcriptomics Reveals Reprogramming of the Lipid Metabolism and Composition in Clear Cell Renal Cell Carcinoma. Metabolites 2020, 10(12).
- Eberlé D, Hegarty B, Bossard P, Ferré P, Foufelle F: SREBP transcription factors: master regulators of lipid homeostasis. Biochimie 2004, 86(11): 839-848.
- Gosmain Y, Dif N, Berbe V, Loizon E, Rieusset J, Vidal H, Lefai E: Regulation of SREBP-1 expression and transcriptional action on HKII and FAS genes during fasting and refeeding in rat tissues. J Lipid Res 2005, 46(4): 697-705.
- Awad NS, Paul V, AlSawaftah NM, Husseini GA: Effect of phospholipid head group on ultrasound-triggered drug release and cellular uptake of immunoliposomes. Sci Rep 2023, 13(1): 16644.
- Duez J, Holleran JP, Ndour PA, Loganathan S, Amireault P, Français O, El Nemer W, Le Pioufle B, Amado IF, Garcia S et al: Splenic retention of Plasmodium falciparum gametocytes to block the transmission of malaria. Antimicrob Agents Chemother 2015, 59(7): 4206-4214.
- Chen C, Zhao W, Lu X, Ma Y, Zhang P, Wang Z, Cui Z, Xia Q: AUP1 regulates lipid metabolism and induces lipid accumulation to accelerate the progression of renal clear cell carcinoma. Cancer Sci 2022, 113(8): 2600-2615.
- Zhang X, Li X: Abnormal Iron and Lipid Metabolism Mediated Ferroptosis in Kidney Diseases and Its Therapeutic Potential. Metabolites 2022, 12(1).
- Chakraborty S, Balan M, Sabarwal A, Choueiri TK, Pal S: Metabolic reprogramming in renal cancer: Events of a metabolic disease. Biochim Biophys Acta Rev Cancer 2021, 1876(1): 188559.
Annals of urologic oncology
p-ISSN: 2617-7765, e-ISSN: 2617-7773
Copyright © Ann Urol Oncol. This work is licensed under a Creative Commons Attribution-NonCommercial-No Derivatives 4.0 International (CC BY-NC-ND 4.0) License.