Review Article | Open Access
Immunotherapies to Nano-Immunotherapies: Advances in Immune Targeting in Bladder Cancer
Beatriz Ramos1, Dakota Rogers21Department of Medical Laboratory Science, University of Kentucky, Lexington 40506, Kentucky, USA.
2School of Pharmaceutical Sciences, University of Kentucky, Lexington 40506, Kentucky, USA.
Correspondence: Dakota Rogers (School of Pharmaceutical Sciences, University of Kentucky, Lexington 40506, Kentucky, USA; Email: dakotauok12@gmail.com).
Annals of Urologic Oncology 2025, 8(1): 1-11. https://doi.org/10.32948/auo.2025.02.01
Received: 18 Nov 2024 | Accepted: 29 Jan 2025 | Published online: 05 Feb 2025
Key words bladder cancer, nano-immunotherapies, BCG immunotherapy, nanovaccines, checkpoint molecules
In terms of adaptive immune responses, recent research in both human and mouse models has uncovered specific actions of various T cell subsets, most of which seem to negatively impact the host, such as insufficient elimination of cancer cells, and/or increased inflammation [32]. Extensive research has been conducted to characterize T cell-mediated immune responses in bladder cancer models, with the objective of advancing targeted immunotherapeutic strategies. A diverse array of T cell subsets has been identified, each playing distinct immunological roles in bladder cancer. These include cytotoxic CD8+ T cells, which exert pro-inflammatory and antitumor effects, regulatory T cells (Tregs) that contribute to immunosuppression, and CD4+ helper T (Th) cells, with a notable presence of Th1-polarized subsets [33]. This heterogeneity in T cell populations within bladder tumors underscores the complexity of immune interactions shaping disease progression and therapeutic outcomes [34]. In the tumor microenvironment, T-cells release IFN-γ, which enhances antigen presentation by dendritic cells via CD40/CD40L, promotes cytotoxic T cell function, and shifts macrophages to an M1 pro-inflammatory state [34]. In bladder cancer murine models, an increase in IFN-γ producing Th1 cells infiltration has been observed. Moreover, neutralizing IFN-γ nullifies the anti-tumor effects of the therapy, highlighting the crucial role of Th1 cells [35]. An elevated density of CD4+ T cells in the tumor has been linked to poor prognosis in NMIBC [36, 37]. Factors released within the tumor microenvironment play a significant role in attracting Tregs from the bloodstream, which subsequently weakens tumor immune surveillance. This immunosuppressive effect is primarily mediated through the secretion of IL-10 and TGF-β [34, 38], which contribute to dampening anti-tumor immune responses. Additionally, these factors may facilitate the depletion or functional impairment of key anti-tumor effector cells and antigen-presenting cells, further weakening immune surveillance [34]. This adaptive shift in T cell phenotype exacerbates immunosuppression, ultimately hindering the activation of nascent anti-tumor immunity and promoting tumor progression. T cells upregulate the expression of programmed cell death protein 1 (PD-1) upon activation, which interacts with its PD-L1 present on the majority of tumor cells, thus impairing the T cells' anti-tumor functions by restricting their effector activities [39].
Immunotherapy works by activating immune system, and has thus become a viable option as a first-line treatment [46, 47] or as part of combination therapy strategies alongside other therapeutic modalities [48]. Immune checkpoint inhibitors aim to boost immune defenses against cancer. This approach leads to improved cancer cell eradication and the establishment of durable anti-tumor immunity [49]. These advancements have significantly broadened the scope of immunotherapy, offering new hope for patients with various malignancies. Although clinical outcomes have been somewhat limited, ongoing clinical trials and experimental models are investigating novel approaches to amplify anti-tumor T cell responses. These include the use of monoclonal antibodies that prevent PD-1’s interaction with PD-L1, thereby improving T cell activity against tumors [39, 50]. The most significant breakthroughs over the past decade has been the development of immune checkpoint inhibitors targeting CTLA-4 and PD-1/PD-L1 [49, 51]. Additionally, other immune checkpoint molecules like tumor necrosis factor receptor 2 (TNFR2) [52], are also being targeted to further modulate T lymphocyte function and improve therapeutic efficacy. The success of immunotherapy with immune checkpoint inhibitors largely depends on how responsive the tumor is to these inhibitors, with factors such as the genomic diversity of the tumor, host germline genetics, microbiome composition, and PD-L1 expression levels influencing their effectiveness [53]. Different immunotherapies being used and tested as bladder cancer treatment are discussed in the following sections in detail (Figure 1).
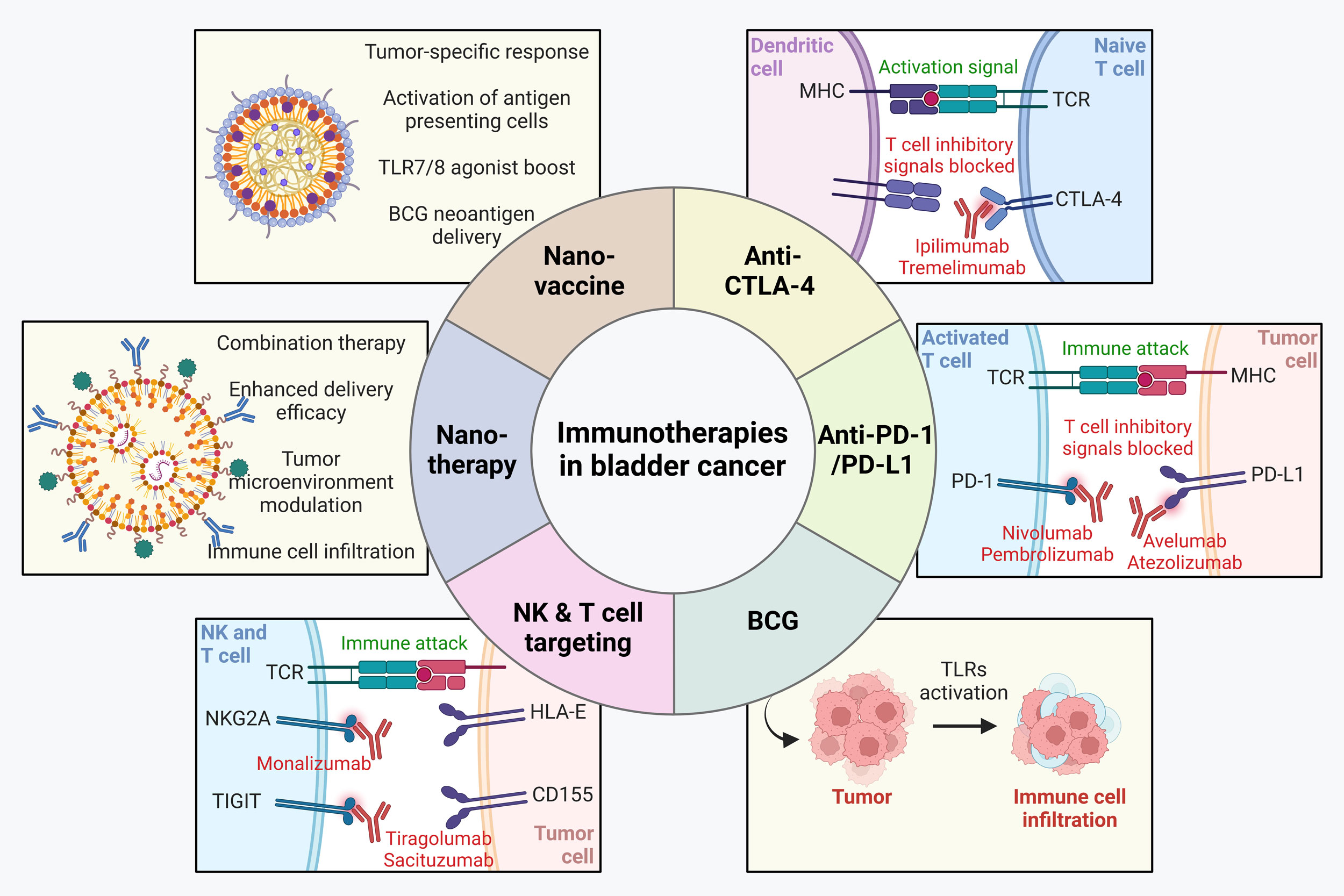
Table 1. Immunotherapies in bladder cancer. |
|||||
Drug / Approach |
Target |
Clinical trials |
Key findings |
FDA approval status |
References |
Ipilimumab |
CTLA-4 |
CheckMate 032, CheckMate 901 |
Combination with nivolumab shows improved response |
Not approved yet for bladder cancer |
[55, 56] |
Tremelimumab |
CTLA-4 |
DANUBE, NCT0281242 |
Combination with durvalumab did not improve survival over chemotherapy |
Not yet approved |
[57, 58] |
Nivolumab |
PD-1 |
CheckMate 275, CheckMate 274 |
Improved survival in platinum-resistant and high-risk MIBC patients |
Approved for metastatic bladder cancer and adjuvant therapy in MIBC |
[59-62] |
Pembrolizumab |
PD-1 |
KEYNOTE-052, KEYNOTE-045 |
Significant response in platinum-refractory patients |
Approved for advanced or metastatic bladder cancer |
[63-65] |
Avelumab |
PD-L1 |
JAVELIN Solid Tumor, JAVELIN Bladder 100 |
Improved disease control in maintenance therapy after chemotherapy |
Approved for maintenance therapy in advanced bladder cancer |
[66-68] |
Atezolizumab |
PD-L1 |
IMvigor130 |
Effective as a first-line option for cisplatin-ineligible patients |
Approved but later restricted due to trial findings |
[69] |
BCG |
Immune activation via TLR2, TLR4, TLR9 |
- |
Standard therapy for NMIBC, induces T cell and NK cell responses |
Approved for NMIBC |
[73, 74] |
Monalizumab |
NKG2A |
COAST, ENHANCE |
Blocks NK cell inhibition, under investigation for combination therapy |
Not yet approved |
[91, 92] |
Tiragolumab |
TIGIT |
NCT05394337 |
Tested in combination with PD-1 inhibitors (atezolizumab) for urothelial carcinoma |
Not yet approved |
[93] |
Sacituzumab |
TIGIT |
NCT03547973 |
Shown to improve survival in metastatic bladder cancer |
Approved for metastatic bladder cancer |
[94, 95] |
KIR2DL5 Inhibitors |
KIR2DL5/CD155 |
Preclinical studies |
Blocking KIR2DL5 enhances NK cell activity |
Not yet approved |
[96] |
Table 2. Preclinical nanoimmunotherapies and nanovaccines in bladder cancer. |
|||
Approach |
Target / Mechanism |
Key findings |
References |
Macrophage-derived exosome-mimetic nanovesicles (EMVs) with AB680 + PD-L1 inhibitor |
CD73 inhibition + PD-L1 blockade |
Enhanced T cell activation and tumor infiltration |
[102, 103] |
Reactive oxygen species (ROS)-sensitive nanoparticles (NP@ESCu) with cuproptosis induction |
Copper ion release + PD-L1 upregulation |
Induced cancer cell death and improved immune response |
[104] |
Gold nanoparticles (GNPs) conjugated with listeriolysin O peptide (GNP-LLO 91–99 nanovaccine) |
Tumor microenvironment modulation |
Increased cytotoxic T cell infiltration and improved response to checkpoint inhibitors |
[105] |
MNC-ICG-NIG@SiO2 (MINS) macrophage-targeted delivery system |
BCG therapy enhancement + autoimmunity modulation |
Improved BCG therapy response through cytokine regulation |
[107] |
TLR7/8 agonist-loaded polymeric nanoparticles |
Dendritic cell activation |
Enhanced CD8+ T cell response and reduced metastasis |
[108, 113, 114] |
BCG cell wall skeleton-based nanovaccine with neoantigens (M27, M30) |
Cancer-specific immune activation |
60% tumor elimination and synergy with PD-L1 inhibitors |
[115] |
None.
Ethical policy
Non applicable.
Availability of data and materials
All data generated or analysed during this study are included in this publication.
Author contributions
BR and DR searched academic literature, wrote the draft manuscript, drew the figures and submitted the final manuscript online.
Competing interests
None.
Funding
None.
- Siegel RL, Miller KD: Cancer statistics, 2023. CA Cancer J Clin 2023, 73(1): 17-48.
- Saginala K, Barsouk A, Aluru JS, Rawla P, Padala SA, Barsouk A: Epidemiology of Bladder Cancer. Med Sci (Basel) 2020, 8(1).
- Witjes JA: Follow-up in non-muscle invasive bladder cancer: facts and future. World J Urol 2021, 39(11): 4047-4053.
- De Santis M, Bellmunt J, Mead G, Kerst JM, Leahy M, Maroto P, Gil T, Marreaud S, Daugaard G, Skoneczna I et al: Randomized phase II/III trial assessing gemcitabine/carboplatin and methotrexate/carboplatin/vinblastine in patients with advanced urothelial cancer who are unfit for cisplatin-based chemotherapy: EORTC study 30986. J Clin Oncol 2012, 30(2): 191-199.
- Okazaki T, Honjo T: The PD-1-PD-L pathway in immunological tolerance. Trends Immunol 2006, 27(4): 195-201.
- Egen JG, Kuhns MS, Allison JP: CTLA-4: new insights into its biological function and use in tumor immunotherapy. Nat Immunol 2002, 3(7): 611-618.
- Salomé B, Sfakianos JP, Ranti D, Daza J, Bieber C, Charap A, Hammer C, Banchereau R, Farkas AM, Ruan DF et al: NKG2A and HLA-E define an alternative immune checkpoint axis in bladder cancer. Cancer Cell 2022, 40(9): 1027-1043.e1029.
- Gavas S, Quazi S, Karpiński TM: Nanoparticles for Cancer Therapy: Current Progress and Challenges. Nanoscale Res Lett 2021, 16(1): 173.
- Joudeh N, Linke D: Nanoparticle classification, physicochemical properties, characterization, and applications: a comprehensive review for biologists. J Nanobiotechnology 2022, 20(1): 262.
- Golombek SK, May JN, Theek B, Appold L, Drude N, Kiessling F, Lammers T: Tumor targeting via EPR: Strategies to enhance patient responses. Adv Drug Deliv Rev 2018, 130: 17-38.
- Handa M, Beg S, Shukla R, Barkat MA, Choudhry H, Singh KK: Recent advances in lipid-engineered multifunctional nanophytomedicines for cancer targeting. J Control Release 2021, 340: 48-59.
- Ashrafizadeh M, Zarrabi A: (Nano)platforms in bladder cancer therapy: Challenges and opportunities. Bioeng Transl Med 2023, 8(1): e10353.
- Amreddy N, Babu A, Muralidharan R, Panneerselvam J, Srivastava A, Ahmed R, Mehta M, Munshi A, Ramesh R: Recent Advances in Nanoparticle-Based Cancer Drug and Gene Delivery. Adv Cancer Res 2018, 137: 115-170.
- Joseph M, Enting D: Immune Responses in Bladder Cancer-Role of Immune Cell Populations, Prognostic Factors and Therapeutic Implications. Front Oncol 2019, 9: 1270.
- Allegrezza MJ, Conejo-Garcia JR: Targeted Therapy and Immunosuppression in the Tumor Microenvironment. Trends Cancer 2017, 3(1): 19-27.
- Ayari C, LaRue H, Hovington H, Caron A, Bergeron A, Têtu B, Fradet V, Fradet Y: High level of mature tumor-infiltrating dendritic cells predicts progression to muscle invasion in bladder cancer. Hum Pathol 2013, 44(8): 1630-1637.
- Basak U, Sarkar T, Mukherjee S, Chakraborty S, Dutta A, Dutta S, Nayak D, Kaushik S, Das T, Sa G: Tumor-associated macrophages: an effective player of the tumor microenvironment. Front Immunol 2023, 14: 1295257.
- Murray PJ, Allen JE, Biswas SK, Fisher EA, Gilroy DW, Goerdt S, Gordon S, Hamilton JA, Ivashkiv LB, Lawrence T et al: Macrophage activation and polarization: nomenclature and experimental guidelines. Immunity 2014, 41(1): 14-20.
- Noy R, Pollard JW: Tumor-associated macrophages: from mechanisms to therapy. Immunity 2014, 41(1): 49-61.
- Biswas SK, Mantovani A: Macrophage plasticity and interaction with lymphocyte subsets: cancer as a paradigm. Nat Immunol 2010, 11(10): 889-896.
- Leblond MM, Zdimerova H, Desponds E, Verdeil G: Tumor-Associated Macrophages in Bladder Cancer: Biological Role, Impact on Therapeutic Response and Perspectives for Immunotherapy. Cancers (Basel) 2021, 13(18): 4712.
- Cao Y, Wang X, Jin T, Tian Y, Dai C, Widarma C, Song R, Xu F: Immune checkpoint molecules in natural killer cells as potential targets for cancer immunotherapy. Signal Transduct Target Ther 2020, 5(1): 250.
- Choucair K, Duff JR, Cassidy CS, Albrethsen MT, Kelso JD, Lenhard A, Staats H, Patel R, Brunicardi FC, Dworkin L et al: Natural killer cells: a review of biology, therapeutic potential and challenges in treatment of solid tumors. Future Oncol 2019, 15(26): 3053-3069.
- Gonzalez H, Hagerling C, Werb Z: Roles of the immune system in cancer: from tumor initiation to metastatic progression. Genes Dev 2018, 32(19-20): 1267-1284.
- Tsujihashi H, Matsuda H, Uejima S, Akiyama T, Kurita T: Role of natural killer cells in bladder tumor. Eur Urol 1989, 16(6):444-449.
- Krpina K, Babarović E, Ðordević G, Markić D, Maricić A, Jonjić N: Impact of NK cell count on bladder cancer recurrence. Urologia 2014, 81(4): 233-236.
- Shaul ME, Fridlender ZG: Cancer-related circulating and tumor-associated neutrophils - subtypes, sources and function. Febs j 2018, 285(23): 4316-4342.
- Vartolomei MD, Porav-Hodade D, Ferro M, Mathieu R, Abufaraj M, Foerster B, Kimura S, Shariat SF: Prognostic role of pretreatment neutrophil-to-lymphocyte ratio (NLR) in patients with non-muscle-invasive bladder cancer (NMIBC): A systematic review and meta-analysis. Urol Oncol 2018, 36(9): 389-399.
- Liu K, Zhao K, Wang L, Sun E: The prognostic values of tumor-infiltrating neutrophils, lymphocytes and neutrophil/lymphocyte rates in bladder urothelial cancer. Pathol Res Pract 2018, 214(8): 1074-1080.
- Masucci MT, Minopoli M, Carriero MV: Tumor Associated Neutrophils. Their Role in Tumorigenesis, Metastasis, Prognosis and Therapy. Front Oncol 2019, 9: 1146.
- Dumitru CA, Lang S, Brandau S: Modulation of neutrophil granulocytes in the tumor microenvironment: mechanisms and consequences for tumor progression. Semin Cancer Biol 2013, 23(3): 141-148.
- Wu J, Abraham SN: The Roles of T cells in Bladder Pathologies. Trends Immunol 2021, 42(3): 248-260.
- Cresswell J, Robertson H, Neal DE, Griffiths TR, Kirby JA: Distribution of lymphocytes of the alpha(E)beta(7) phenotype and E-cadherin in normal human urothelium and bladder carcinomas. Clin Exp Immunol 2001, 126(3): 397-402.
- Oh DY, Kwek SS, Raju SS, Li T, McCarthy E, Chow E, Aran D, Ilano A, Pai CS, Rancan C et al: Intratumoral CD4(+) T Cells Mediate Anti-tumor Cytotoxicity in Human Bladder Cancer. Cell 2020, 181(7): 1612-1625.e1613.
- Sato Y, Bolzenius JK, Eteleeb AM, Su X, Maher CA, Sehn JK, Arora VK: CD4+ T cells induce rejection of urothelial tumors after immune checkpoint blockade. JCI Insight 2018, 3(23): e121062.
- Zhang Q, Hao C, Cheng G, Wang L, Wang X, Li C, Qiu J, Ding K: High CD4⁺ T cell density is associated with poor prognosis in patients with non-muscle-invasive bladder cancer. Int J Clin Exp Pathol 2015, 8(9): 11510-11516.
- Shi MJ, Meng XY, Wu QJ, Zhou XH: High CD3D/CD4 ratio predicts better survival in muscle-invasive bladder cancer. Cancer Manag Res 2019, 11: 2987-2995.
- Tay RE, Richardson EK, Toh HC: Revisiting the role of CD4(+) T cells in cancer immunotherapy-new insights into old paradigms. Cancer Gene Ther 2021, 28(1-2): 5-17.
- Han Y, Liu D, Li L: PD-1/PD-L1 pathway: current researches in cancer. Am J Cancer Res 2020, 10(3): 727-742.
- Crispen PL, Kusmartsev S: Mechanisms of immune evasion in bladder cancer. Cancer Immunol Immunother 2020, 69(1): 3-14.
- Rouanne M, Adam J, Radulescu C, Letourneur D, Bredel D, Mouraud S, Goubet AG, Leduc M, Chen N, Tan TZ et al: BCG therapy downregulates HLA-I on malignant cells to subvert antitumor immune responses in bladder cancer. J Clin Invest 2022, 132(12): e145666.
- Rouanne M, Roumiguié M, Houédé N, Masson-Lecomte A, Colin P, Pignot G, Larré S, Xylinas E, Rouprêt M, Neuzillet Y: Development of immunotherapy in bladder cancer: present and future on targeting PD(L)1 and CTLA-4 pathways. World J Urol 2018, 36(11): 1727-1740.
- Maas M, Hilsendecker A, Pertoll A, Stühler V, Walz S, Rausch S: PD-L1 Expression in High-Risk Non-Muscle-Invasive Bladder Cancer Is Influenced by Intravesical Bacillus Calmette-Guérin (BCG) Therapy. Cancers (Basel) 2024, 16(7): 1356.
- Masson D, Jarry A, Baury B, Blanchardie P, Laboisse C, Lustenberger P, Denis MG: Overexpression of the CD155 gene in human colorectal carcinoma. Gut 2001, 49(2): 236-240.
- Triki H, Charfi S, Bouzidi L, Ben Kridis W, Daoud J, Chaabane K, Sellami-Boudawara T, Rebai A, Cherif B: CD155 expression in human breast cancer: Clinical significance and relevance to natural killer cell infiltration. Life Sci 2019, 231: 116543.
- Akkın S, Varan G, Bilensoy E: A Review on Cancer Immunotherapy and Applications of Nanotechnology to Chemoimmunotherapy of Different Cancers. Molecules 2021, 26(11): 3382.
- Jagodinsky JC, Harari PM, Morris ZS: The Promise of Combining Radiation Therapy With Immunotherapy. Int J Radiat Oncol Biol Phys 2020, 108(1): 6-16.
- Merino M, Contreras A, Casares N, Troconiz IF, Ten Hagen TL, Berraondo P, Zalba S, Garrido MJ: A new immune-nanoplatform for promoting adaptive antitumor immune response. Nanomedicine 2019, 17: 13-25.
- Robert C: A decade of immune-checkpoint inhibitors in cancer therapy. Nat Commun 2020, 11(1): 3801.
- Acúrcio RC, Pozzi S, Carreira B, Pojo M, Gómez-Cebrián N, Casimiro S, Fernandes A, Barateiro A, Farricha V, Brito J et al: Therapeutic targeting of PD-1/PD-L1 blockade by novel small-molecule inhibitors recruits cytotoxic T cells into solid tumor microenvironment. J Immunother Cancer 2022, 10(7): e004695.
- Waldman AD, Fritz JM, Lenardo MJ: A guide to cancer immunotherapy: from T cell basic science to clinical practice. Nat Rev Immunol 2020, 20(11): 651-668.
- Tam EM, Fulton RB: Antibody-mediated targeting of TNFR2 activates CD8(+) T cells in mice and promotes antitumor immunity. Sci Transl Med 2019, 11(512): eaax0720.
- Havel JJ, Chowell D, Chan TA: The evolving landscape of biomarkers for checkpoint inhibitor immunotherapy. Nat Rev Cancer 2019, 19(3): 133-150.
- Traynor K: Ipilimumab approved for metastatic melanoma. Am J Health Syst Pharm 2011, 68(9): 768.
- Sharma P, Siefker-Radtke A, de Braud F, Basso U, Calvo E, Bono P, Morse MA, Ascierto PA, Lopez-Martin J, Brossart P et al: Nivolumab Alone and With Ipilimumab in Previously Treated Metastatic Urothelial Carcinoma: CheckMate 032 Nivolumab 1 mg/kg Plus Ipilimumab 3 mg/kg Expansion Cohort Results. J Clin Oncol 2019, 37(19): 1608-1616.
- van der Heijden MS, Sonpavde G, Powles T, Necchi A, Burotto M, Schenker M, Sade JP, Bamias A, Beuzeboc P, Bedke J et al: Nivolumab plus Gemcitabine-Cisplatin in Advanced Urothelial Carcinoma. N Engl J Med 2023, 389(19): 1778-1789.
- Powles T, van der Heijden MS, Castellano D, Galsky MD, Loriot Y, Petrylak DP, Ogawa O, Park SH, Lee JL, De Giorgi U et al: Durvalumab alone and durvalumab plus tremelimumab versus chemotherapy in previously untreated patients with unresectable, locally advanced or metastatic urothelial carcinoma (DANUBE): a randomised, open-label, multicentre, phase 3 trial. Lancet Oncol 2020, 21(12): 1574-1588.
- Gao J, Navai N, Alhalabi O: Neoadjuvant PD-L1 plus CTLA-4 blockade in patients with cisplatin-ineligible operable high-risk urothelial carcinoma. Nat Med 2020, 26(12): 1845-1851.
- Sharma P, Retz M, Siefker-Radtke A, Baron A, Necchi A, Bedke J, Plimack ER, Vaena D, Grimm MO, Bracarda S et al: Nivolumab in metastatic urothelial carcinoma after platinum therapy (CheckMate 275): a multicentre, single-arm, phase 2 trial. Lancet Oncol 2017, 18(3): 312-322.
- Sharma P, Callahan MK, Bono P, Kim J, Spiliopoulou P, Calvo E, Pillai RN, Ott PA, de Braud F, Morse M et al: Nivolumab monotherapy in recurrent metastatic urothelial carcinoma (CheckMate 032): a multicentre, open-label, two-stage, multi-arm, phase 1/2 trial. Lancet Oncol 2016, 17(11): 1590-1598.
- Galsky MD, Saci A, Szabo PM, Han GC, Grossfeld G, Collette S, Siefker-Radtke A, Necchi A, Sharma P: Nivolumab in Patients with Advanced Platinum-resistant Urothelial Carcinoma: Efficacy, Safety, and Biomarker Analyses with Extended Follow-up from CheckMate 275. Clin Cancer Res 2020, 26(19): 5120-5128.
- Bajorin DF, Witjes JA, Gschwend JE, Schenker M, Valderrama BP, Tomita Y, Bamias A, Lebret T, Shariat SF, Park SH et al: Adjuvant Nivolumab versus Placebo in Muscle-Invasive Urothelial Carcinoma. N Engl J Med 2021, 384(22): 2102-2114.
- Balar AV, Castellano D, O'Donnell PH, Grivas P, Vuky J, Powles T, Plimack ER, Hahn NM, de Wit R, Pang L et al: First-line pembrolizumab in cisplatin-ineligible patients with locally advanced and unresectable or metastatic urothelial cancer (KEYNOTE-052): a multicentre, single-arm, phase 2 study. Lancet Oncol 2017, 18(11): 1483-1492.
- Tree AC, Jones K, Hafeez S, Sharabiani MTA, Harrington KJ, Lalondrelle S, Ahmed M, Huddart RA: Dose-limiting Urinary Toxicity With Pembrolizumab Combined With Weekly Hypofractionated Radiation Therapy in Bladder Cancer. Int J Radiat Oncol Biol Phys 2018, 101(5): 1168-1171.
- Huddart R, Jones K, Bucinskaite G, Pillai S, Tree A, Greenlay E, Potts L, Hafeez S: Phase 1 trial of Hypofractionated radiotherapy and Pembrolizumab in the treatment of locally advanced or metastatic bladder cancer: Results of dose escalation phase of the PLUMMB trial (NCT02560636). Eur Urol Open Sci 2022, 45: S242-S243.
- Apolo A, Ellerton J, Infante J, Agrawal M, Gordon M, Aljumaily R, Britten C, Dirix L, Lee K, Taylor M: Avelumab treatment of metastatic urothelial carcinoma (mUC) in the phase 1b JAVELIN solid Tumor study: updated analysis with≥ 6 months of follow-up in all patients. Ann Oncol 2017, 28: v300-v301.
- Collins JM, Gulley JL: Product review: avelumab, an anti-PD-L1 antibody. Hum Vaccin Immunother 2019, 15(4): 891-908.
- Powles T, Park SH, Voog E, Caserta C, Valderrama BP, Gurney H, Kalofonos H, Radulović S, Demey W, Ullén A et al: Avelumab Maintenance Therapy for Advanced or Metastatic Urothelial Carcinoma. N Engl J Med 2020, 383(13): 1218-1230.
- Suzman DL, Agrawal S, Ning YM, Maher VE, Fernandes LL, Karuri S, Tang S, Sridhara R, Schroeder J, Goldberg KB et al: FDA Approval Summary: Atezolizumab or Pembrolizumab for the Treatment of Patients with Advanced Urothelial Carcinoma Ineligible for Cisplatin-Containing Chemotherapy. Oncologist 2019, 24(4): 563-569.
- Massard C, Gordon MS, Sharma S, Rafii S, Wainberg ZA, Luke J, Curiel TJ, Colon-Otero G, Hamid O, Sanborn RE et al: Safety and Efficacy of Durvalumab (MEDI4736), an Anti-Programmed Cell Death Ligand-1 Immune Checkpoint Inhibitor, in Patients With Advanced Urothelial Bladder Cancer. J Clin Oncol 2016, 34(26): 3119-3125.
- Powles T, O'Donnell PH, Massard C, Arkenau HT, Friedlander TW, Hoimes CJ, Lee JL, Ong M, Sridhar SS, Vogelzang NJ et al: Efficacy and Safety of Durvalumab in Locally Advanced or Metastatic Urothelial Carcinoma: Updated Results From a Phase 1/2 Open-label Study. JAMA Oncol 2017, 3(9): e172411.
- Morales A, Eidinger D, Bruce AW: Intracavitary Bacillus Calmette-Guerin in the treatment of superficial bladder tumors. J Urol 1976, 116(2): 180-183.
- Pettenati C, Ingersoll MA: Mechanisms of BCG immunotherapy and its outlook for bladder cancer. Nat Rev Urol 2018, 15(10): 615-625.
- Sylvester RJ, van der Meijden AP, Witjes JA, Kurth K: Bacillus calmette-guerin versus chemotherapy for the intravesical treatment of patients with carcinoma in situ of the bladder: a meta-analysis of the published results of randomized clinical trials. J Urol 2005, 174(1): 86-91; discussion 91-82.
- Zhao W, Schorey JS, Bong-Mastek M, Ritchey J, Brown EJ, Ratliff TL: Role of a bacillus Calmette-Guérin fibronectin attachment protein in BCG-induced antitumor activity. Int J Cancer 2000, 86(1): 83-88.
- Bevers RF, de Boer EC, Kurth KH, Schamhart DH: BCG-induced interleukin-6 upregulation and BCG internalization in well and poorly differentiated human bladder cancer cell lines. Eur Cytokine Netw 1998, 9(2): 181-186.
- Thiel T, Ryk C, Chatzakos V, Hallén Grufman K, Bavand-Chobot N, Flygare J, Wiklund NP, de Verdier PJ: Secondary stimulation from Bacillus Calmette-Guérin induced macrophages induce nitric oxide independent cell-death in bladder cancer cells. Cancer Lett 2014, 348(1-2): 119-125.
- Zhang Y, Zhang Z: The history and advances in cancer immunotherapy: understanding the characteristics of tumor-infiltrating immune cells and their therapeutic implications. Cell Mol Immunol 2020, 17(8): 807-821.
- Brightbill HD, Libraty DH, Krutzik SR, Yang RB, Belisle JT, Bleharski JR, Maitland M, Norgard MV, Plevy SE, Smale ST et al: Host defense mechanisms triggered by microbial lipoproteins through toll-like receptors. Science 1999, 285(5428): 732-736.
- De Boer EC, De Jong WH, Van Der Meijden AP, Steerenberg PA, Witjes JA, Vegt PD, Debruyne FM, Ruitenberg EJ: Presence of activated lymphocytes in the urine of patients with superficial bladder cancer after intravesical immunotherapy with bacillus Calmette-Guérin. Cancer Immunol Immunother 1991, 33(6): 411-416.
- Prescott S, James K, Hargreave TB, Chisholm GD, Smyth JF: Intravesical Evans strain BCG therapy: quantitative immunohistochemical analysis of the immune response within the bladder wall. J Urol 1992, 147(6): 1636-1642.
- Pryor K, Goddard J, Goldstein D, Stricker P, Russell P, Golovsky D, Penny R: Bacillus Calmette-Guerin (BCG) enhances monocyte- and lymphocyte-mediated bladder tumour cell killing. Br J Cancer 1995, 71(4): 801-807.
- Suriano F, Santini D, Perrone G, Amato M, Vincenzi B, Tonini G, Muda A, Boggia S, Buscarini M, Pantano F: Tumor associated macrophages polarization dictates the efficacy of BCG instillation in non-muscle invasive urothelial bladder cancer. J Exp Clin Cancer Res 2013, 32(1): 87.
- Pichler R, Fritz J, Zavadil C, Schäfer G, Culig Z, Brunner A: Tumor-infiltrating immune cell subpopulations influence the oncologic outcome after intravesical Bacillus Calmette-Guérin therapy in bladder cancer. Oncotarget 2016, 7(26): 39916-39930.
- Fan Z, Deng J, Wang Y, Fan X, Xie J: Bladder Cancer: Immunotherapy and Pelvic Lymph Node Dissection. Vaccines (Basel) 2024, 12(2): 150.
- Kleinnijenhuis J, Quintin J, Preijers F, Joosten LA, Jacobs C, Xavier RJ, van der Meer JW, van Crevel R, Netea MG: BCG-induced trained immunity in NK cells: Role for non-specific protection to infection. Clin Immunol 2014, 155(2): 213-219.
- García-Cuesta EM, Esteso G, Ashiru O, López-Cobo S, Álvarez-Maestro M, Linares A, Ho MM, Martínez-Piñeiro L, H TR, Valés-Gómez M: Characterization of a human anti-tumoral NK cell population expanded after BCG treatment of leukocytes. Oncoimmunology 2017, 6(4): e1293212.
- Guillamón CF, Gimeno L, Server G, Martínez-Sánchez MV, Escudero JF, López-Cubillana P, Cabezas-Herrera J, Campillo JA, Abellan DJ, Martínez-García J et al: Immunological Risk Stratification of Bladder Cancer Based on Peripheral Blood Natural Killer Cell Biomarkers. Eur Urol Oncol 2021, 4(2): 246-255.
- Seliger B, Jasinski-Bergner S, Quandt D, Stoehr C, Bukur J, Wach S, Legal W, Taubert H, Wullich B, Hartmann A: HLA-E expression and its clinical relevance in human renal cell carcinoma. Oncotarget 2016, 7(41): 67360-67372.
- Borst L, van der Burg SH: The NKG2A-HLA-E Axis as a Novel Checkpoint in the Tumor Microenvironment. Clin Cancer Res 2020, 26(21): 5549-5556.
- Herbst RS, Majem M, Barlesi F, Carcereny E, Chu Q: COAST: An Open-Label, Phase II, Multidrug Platform Study of Durvalumab Alone or in Combination With Oleclumab or Monalizumab in Patients With Unresectable, Stage III Non-Small-Cell Lung Cancer. J Clin Oncol 2022, 40(29): 3383-3393.
- van Hall T, André P, Horowitz A, Ruan DF, Borst L, Zerbib R, Narni-Mancinelli E, van der Burg SH, Vivier E: Monalizumab: inhibiting the novel immune checkpoint NKG2A. J Immunother Cancer 2019, 7(1): 263.
- Liu Z, Zeng H, Jin K: TIGIT and PD-1 expression atlas predicts response to adjuvant chemotherapy and PD-L1 blockade in muscle-invasive bladder cancer. Br J Cancer 2022, 126(9): 1310-1317.
- Husain B, Ramani SR, Chiang E, Lehoux I, Paduchuri S, Arena TA, Patel A, Wilson B, Chan P, Franke Y et al: A Platform for Extracellular Interactome Discovery Identifies Novel Functional Binding Partners for the Immune Receptors B7-H3/CD276 and PVR/CD155. Mol Cell Proteomics 2019, 18(11): 2310-2323.
- Zhang J, Zhu Y, Wang Q, Kong Y, Sheng H, Guo J, Xu J, Dai B: Poliovirus receptor CD155 is up-regulated in muscle-invasive bladder cancer and predicts poor prognosis. Urol Oncol 2020, 38(2): 41.e11-41.e18.
- Ren X, Peng M, Xing P, Wei Y, Galbo PM, Jr., Corrigan D, Wang H, Su Y, Dong X, Sun Q et al: Blockade of the immunosuppressive KIR2DL5/PVR pathway elicits potent human NK cell-mediated antitumor immunity. J Clin Invest 2022, 132(22): e163620.
- Liu J, Gao Y, Song C, Liao W, Meng L, Yang S, Xiong Y: Immunotherapeutic prospects and progress in bladder cancer. J Cell Mol Med 2024, 28(5): e18101.
- Okobi TJ, Uhomoibhi TO, Akahara DE, Odoma VA, Sanusi IA, Okobi OE, Umana I, Okobi E, Okonkwo CC, Harry NM: Immune Checkpoint Inhibitors as a Treatment Option for Bladder Cancer: Current Evidence. Cureus 2023, 15(6): e40031.
- Barone B, Calogero A, Scafuri L: Immune Checkpoint Inhibitors as a Neoadjuvant/Adjuvant Treatment of Muscle-Invasive Bladder Cancer: A Systematic Review. Cancers (Basel) 2022, 14(10): 2545.
- Tian Y, Liu Z, Wang J, Li L, Wang F, Zhu Z, Wang X: Nanomedicine for Combination Urologic Cancer Immunotherapy. Pharmaceutics 2023, 15(2): 546.
- Chehelgerdi M, Chehelgerdi M, Allela OQB, Pecho RDC, Jayasankar N, Rao DP, Thamaraikani T, Vasanthan M, Viktor P, Lakshmaiya N et al: Progressing nanotechnology to improve targeted cancer treatment: overcoming hurdles in its clinical implementation. Mol Cancer 2023, 22(1): 169.
- Piovesan D, Tan JBL: Targeting CD73 with AB680 (Quemliclustat), a Novel and Potent Small-Molecule CD73 Inhibitor, Restores Immune Functionality and Facilitates Antitumor Immunity. Mol Cancer Ther 2022, 21(6): 948-959.
- Zhou Q, Ding W, Qian Z, Zhu Q, Sun C, Yu Q, Tai Z: Immunotherapy Strategy Targeting Programmed Cell Death Ligand 1 and CD73 with Macrophage-Derived Mimetic Nanovesicles to Treat Bladder Cancer. Mol Pharm 2021, 18(11): 4015-4028.
- Guo B, Yang F, Zhang L, Zhao Q, Wang W, Yin L, Chen D, Wang M, Han S, Xiao H: Cuproptosis Induced by ROS Responsive Nanoparticles with Elesclomol and Copper Combined with αPD-L1 for Enhanced Cancer Immunotherapy. Adv Mater 2023, 35(22): e2212267.
- Terán-Navarro H, Zeoli A, Salines-Cuevas D, Marradi M: Gold Glyconanoparticles Combined with 91-99 Peptide of the Bacterial Toxin, Listeriolysin O, Are Efficient Immunotherapies in Experimental Bladder Tumors. Cancers (Basel) 2022, 14(10): 2413.
- Zeng S, Xing S, Zhang Y, Wang H, Liu Q: Nano-Bacillus Calmette-Guérin immunotherapies for improved bladder cancer treatment. J Zhejiang Univ Sci B 2024, 25(7): 557-567.
- Guo P, Dai P, Yang S, Wang Z, Tong Z, Hou D, Liu X, Xu W: Engineered Macrophages Tune Intratumoral Cytokines through Precisely Controlled Self-Pyroptosis to Enhance Bladder Cancer Immunotherapy. Small 2024, 20(13): e2306699.
- Kim H, Niu L, Larson P, Kucaba TA, Murphy KA, James BR, Ferguson DM, Griffith TS, Panyam J: Polymeric nanoparticles encapsulating novel TLR7/8 agonists as immunostimulatory adjuvants for enhanced cancer immunotherapy. Biomaterials 2018, 164: 38-53.
- Feng C, Tan P, Nie G, Zhu M: Biomimetic and bioinspired nano-platforms for cancer vaccine development. Exploration (Beijing) 2023, 3(3): 20210263.
- Kaur A, Baldwin J, Brar D, Salunke DB, Petrovsky N: Toll-like receptor (TLR) agonists as a driving force behind next-generation vaccine adjuvants and cancer therapeutics. Curr Opin Chem Biol 2022, 70: 102172.
- Desai N, Chavda V: Cancer Nanovaccines: Nanomaterials and Clinical Perspectives. Small 2024, 20(35): e2401631.
- Paston SJ, Brentville VA, Symonds P, Durrant LG: Cancer Vaccines, Adjuvants, and Delivery Systems. Front Immunol 2021, 12: 627932.
- Kim H, Griffith TS: Poly(d,l-lactide-co-glycolide) Nanoparticles as Delivery Platforms for TLR7/8 Agonist-Based Cancer Vaccine. J Pharmacol Exp Ther 2019, 370(3): 715-724.
- Kim H, Khanna V: TLR7/8 Agonist-Loaded Nanoparticles Augment NK Cell-Mediated Antibody-Based Cancer Immunotherapy. Mol Pharm 2020, 17(6): 2109-2124.
- Liu K, Peng J, Guo Y, Li Y, Qi X, Duan D, Li T, Li J, Niu Y, Han G: Expanding the Potential of Neoantigen Vaccines: Harnessing Bacille Calmette-Guérin Cell-Wall-Based Nanoscale Adjuvants for Enhanced Cancer Immunotherapy. ACS Nano 2024, 18(18): 11910-11920.
- Berraondo P, Sanmamed MF, Ochoa MC, Etxeberria I, Aznar MA, Pérez-Gracia JL, Rodríguez-Ruiz ME, Ponz-Sarvise M, Castañón E, Melero I: Cytokines in clinical cancer immunotherapy. Br J Cancer 2019, 120(1): 6-15.
- Derré L, Lucca I, Cesson V, Bohner P, Crettenand F, Rodrigues-Dias SC, Dartiguenave F, Masnada A, Teixeira-Pereira C, Benmerzoug S et al: Intravesical Ty21a treatment of non-muscle invasive bladder cancer induces immune responses that correlate with safety and may be associated to therapy potential. J Immunother Cancer 2023, 11(12): e008020.
- Zhang P, Wang J, Wang D, Wang H, Shan F, Chen L, Hou Y, Wang E, Lu CL: Dendritic cell vaccine modified by Ag85A gene enhances anti-tumor immunity against bladder cancer. Int Immunopharmacol 2012, 14(3): 252-260.
- Wang S, Wang Y, Liu J, Shao S, Li X, Gao J, Niu H, Wang X: Silencing B7-H1 enhances the anti-tumor effect of bladder cancer antigen-loaded dendritic cell vaccine in vitro. Onco Targets Ther 2014, 7: 1389-1396.
Annals of urologic oncology
p-ISSN: 2617-7765, e-ISSN: 2617-7773
Copyright © Ann Urol Oncol. This work is licensed under a Creative Commons Attribution-NonCommercial-No Derivatives 4.0 International (CC BY-NC-ND 4.0) License.