Review Article | Open Access
Glutamine Metabolism in Prostate Cancer
Xuguang Guo1, 2, 3, 4
1Department of Clinical Laboratory Medicine, The Third Affiliated Hospital of Guangzhou Medical University, Guangzhou, 510150, China.
2Department of Clinical Medicine, The Third Clinical School of Guangzhou Medical University, Guangzhou, 511436, China.
3Key Laboratory for Major Obstetric Diseases of Guangdong Province, The Third Affiliated Hospital of Guangzhou Medical University, Guangzhou, 510150, China.
4Key Laboratory of Reproduction & Genetics of Guangdong Higher Education Institutes, The Third Affiliated Hospital of Guangzhou Medical University, Guangzhou, 510150, China.
Correspondence: Xuguang Guo (Department of Clinical Laboratory Medicine, The Third Affiliated Hospital of Guangzhou Medical University, Guangzhou, 510150, China; Email: gysygxg@gmail.com).
Annals of Urologic Oncology 2024, 7(3): 83-89. https://doi.org/10.32948/auo.2024.08.01
Received: 22 Jul 2024 | Accepted: 01 Aug 2024 | Published online: 04 Aug 2024
Key words metabolic reprogramming, prostate cancer, glutamine metabolism, neuroendocrine, tricarboxylic acid cycle
Unlike normal cells, cancer cells adjust their metabolic pathways to promote growth and survival, leading to different nutritional requirements. For example, the Warburg effect is a change in the metabolism of most neoplastic cells, allowing them to convert glucose into lactate even in the presence of sufficient oxygen, a process commonly known as aerobic glycolysis. This change was initially thought to be due to mitochondrial dysfunction leading to oxidative defects, but studies also show that the rapid uptake and metabolism of glucose enable cancer cells to limit its supply to multiple non-mitochondrial pathways, such as the pentose phosphate pathway, the ribose pathway required for producing ribose and reducing equivalents like (Nicotinamide adenine dinucleotide phosphate, NADPH) for reductive biosynthesis, and the hexose metabolic pathways essential for glycerol used in protein glycosylation and complex lipid synthesis [3, 4]. The Warburg effect may indirectly support synthetic metabolism while maintaining abundant glycolytic intermediates within cells, facilitating involvement in the pentose phosphate pathway and other intracellular biosynthetic pathways [5]. Although the mechanisms behind cancer cell metabolic changes and their impact on cancer behavior remain unclear, there is growing awareness of cancer cells' dependence on specific metabolic pathways and the potential for therapeutic development targeting these dependencies. Therefore, this review provides a brief overview of the glutamine metabolism in prostate cancer.
Normal prostate tissue and prostate tumors exhibit distinct metabolic profiles. Primary prostate cancer tends to increase oxidative phosphorylation while showing limited glycolysis. Fatty acid synthesis also plays an important role in early prostate tumor events and is associated with disease progression [9]. Increased glycolysis is a characteristic of CRPC. Studies indicate a tendency towards glycolysis in advanced PCa cells, with treatment-resistant PCa cells exhibit higher glucose consumption and lactate secretion compared to naive PCa cells [10, 11]. From a mechanistic standpoint, CD44 and Ataxia Telangiectasia Mutated (ATM), proteins involved in vascular dilation and genomic stability, are considered as key regulatory factors. Their alterations significantly impact the glucose metabolism of PCa. Li et al. [12] demonstrated that specific expression of CD44 in neuroendocrine prostate cancer (NEPC) significantly increases the levels of 6-phosphofructo-2-kinase/fructose-2,6-bisphosphatase 4 (PFKFB4). PFKFB4 is one of the rate-limiting enzymes in the glycolysis pathway [13]. Xu et al. [10] demonstrated that ATM mutations (a common genetic event observed in recurrent PCa) upregulate the expression of lactate dehydrogenase-A (LDHA), which is a key enzyme converting pyruvate to lactate. Inhibition of CD44 increases the chemotherapeutic sensitivity of small-cell neuroendocrine cancer [12]. Similarly, targeting LDHA by disrupting the link between ATM alterations and LDHA activation may be a strategy for overcoming PCa resistance to poly (ADP-ribose) polymerase (PARP) inhibitors.
The body's glutamine concentration and availability are influenced by a delicate equilibrium between its synthesis, release, and uptake across various human organs and tissues. Specific organs such as the lungs, liver, brain, skeletal muscles, and adipose tissue exhibit distinctive activities of glutamine synthetase. Conversely, among 31 major tissues that heavily consume glutamine—including the intestinal mucosa, leukocytes, and renal tubular cells—three tissues possess notable levels of glutamine enzyme activity and cofactors that facilitate glutamine degradation. However, the liver may become a site of glutamine consumption under certain conditions, such as reduced intake of carbohydrates [19] and amino acids [20], high catabolic states, illness, and stress [21], leading to decreased glutamine synthesis particularly in the muscle l. Some hormones such as glucocorticoids [22], thyroid hormones [23], growth hormone [24], and insulin [25] can regulate the activity of glutamine metabolism regulatory enzymes.
The intracellular enzymes involved in glutamine metabolism are primarily glutamine synthetase (GS) and phosphate-dependent glutaminase (GLS). GS facilitates the synthesis of glutamine from ammonia (NH4+) and glutamate, requiring ATP as an energy source. In contrast, GLS breaks down glutamine, converting it back into glutamate and NH4+ [26, 27] (Figure 1). GS predominantly resides in the cytoplasm, whereas GLS is primarily localized within the mitochondria. This segregation in cellular localization corresponds with their respective enzymatic roles. Glutamine synthetase produces glutamine to aid in the synthesis of cytoplasmic proteins and nucleotides, while GLS converts glutamine into glutamate. This process is crucial for the tricarboxylic acid cycle and the formation of the metabolic intermediate 2-oxoglutarate [28]. The production of glutamine by GS depends on the availability of glutamate. Glutamate forms from 2-oxoglutarate and NH4+ via glutamate dehydrogenase's catalytic activity and can also arise from the catabolic metabolism of alternative amino acids like branched-chain amino acids (BCAAs) and leucine [20, 29].
Studies have shown that branched-chain amino acids like leucine can readily transaminate with α-ketoglutarate to form glutamate. Glutamate can react with free NH3, and with the help of glutamine synthetase, it is converted into glutamine. [30, 31]. The levels of glutamine in tissues and blood are influenced by the functioning of GS or GLS. During catabolic states like cancer [32], sepsis [33], infections [34], surgical procedures [35], traumatic injuries [36], and prolonged strenuous exercise [37], the body's ability to produce sufficient glutamine internally may be insufficient to meet its requirements. In such deficient circumstances, glutamine serves as a conditionally essential amino acid by stimulating elevated GLS expression and suppressing GS activity. It is important to note that although plasma glutamine levels decrease from 500-800 µmol/L to 300-400 µmol/L, cells dependent on this amino acid actually show little impact on their proliferation and function [38]. Conversely, heightened tissue catabolism leads to diminished glutamine reserves in the body, particularly in muscles and the liver (Figure 2).The reduced glutamine levels in human tissues have systemic implications because this amino acid supplies nitrogen atoms necessary for synthesizing purines, pyrimidines, and amino sugars [39]. Continued high degradation of glutamine in these tissues can disrupt metabolic pathways and mechanisms that heavily depend on glutamine availability, resulting in immune suppression. The research indicates that bacterial infections (like Escherichia coli) can modify their metabolic processes and use glutamine to counteract the impacts of acid stress and copper toxicity [40]. As a result, bacterial pathogens can adjust and thrive by modifying essential antimicrobial strategies imposed by the host within fundamental metabolic pathways.
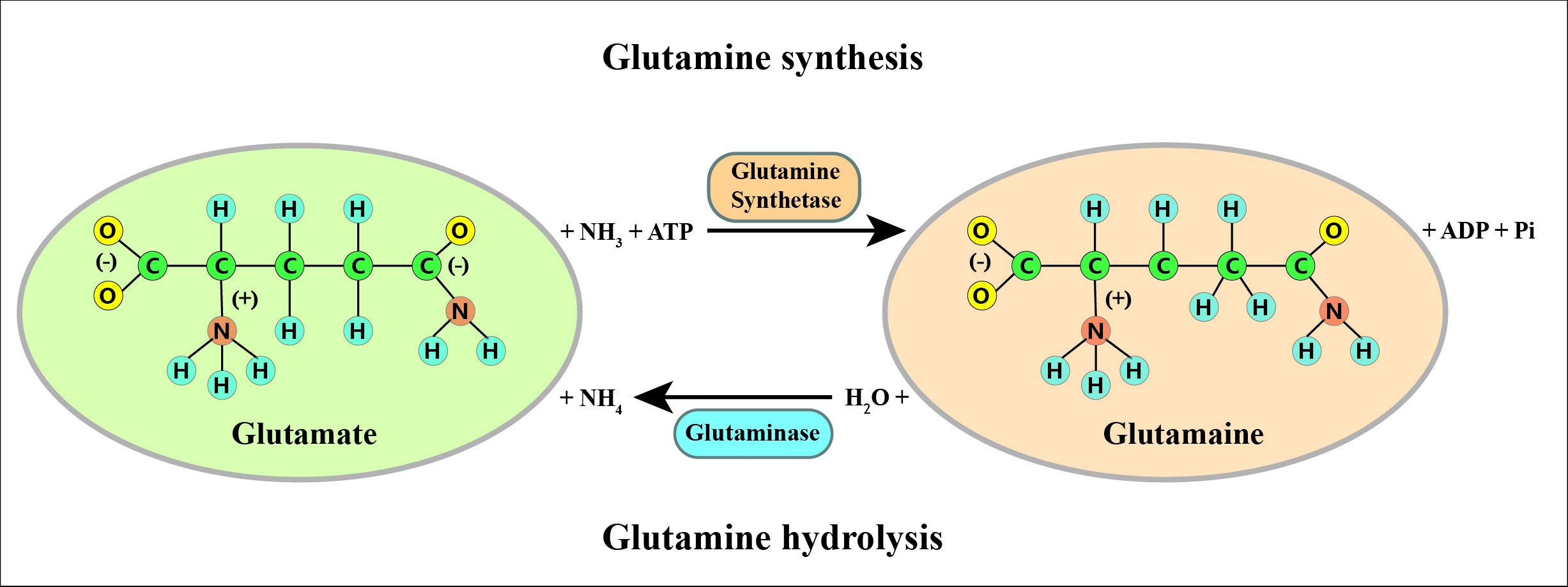
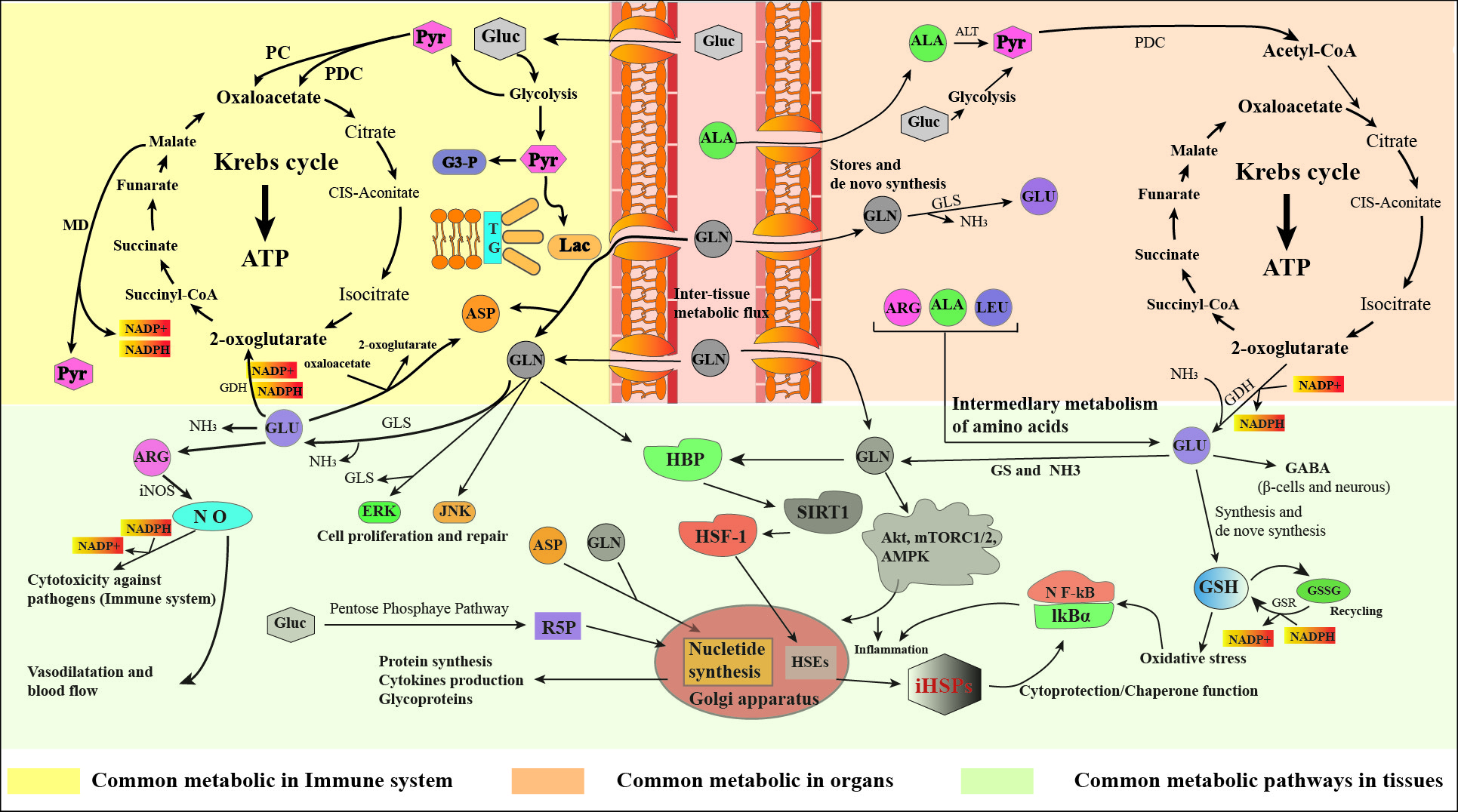
Glutamine's chemical structure not only includes a carbon chain skeleton composed of five carbon atoms but also incorporates two nitrogen atoms. The glutamine metabolism pathway mediated by GLS1 described earlier actually refers specifically to the carbon metabolism of glutamine. In other words, through the catalytic action of GLS1, glutamine is gradually converted into α-ketoglutarate, which then enters the TCA cycle. However, in addition to this, the metabolism of glutamine also includes nitrogen metabolism pathways involving two nitrogen atoms. Specifically, during carbon metabolism, the nitrogen elements of glutamine gradually detach from the carbon chain. However, these nitrogen elements are not merely excreted outside the cell as waste products such as free amines. Instead, they are more involved in the biochemical synthesis of numerous important nitrogen-containing compounds, such as nucleotides and other non-essential amino acids [44, 45]. Therefore, the metabolic network of glutamine is actually regulated by both its carbon and nitrogen metabolic pathways. These two pathways cooperate with each other to ensure the comprehensive utilization of glutamine by tumor cells.
The active metabolic pathways rely on the support of highly active key enzymes. In pyrimidine synthesis, three crucial enzymes are involved: carbamoyl-phosphate synthetase 2, aspartate transcarbamylase, and dihydroorotase (CAD), along with dihydroorotate dehydrogenase (DHODH) and glutamic oxaloacetic transaminase (GOT) [43]. Bioinformatics analysis revealed that, compared to early primary PCa, only the expression level of CAD is significantly elevated in CRPC samples, while the other two key enzymes (DHODH and GOT) show no significant changes in comparison between primary PCa and CRPC samples. Similar results were confirmed in experiments with in cell lines representing CRPC exhibit higher levels of CAD protein. These early experimental results suggest that CAD is likely the most crucial enzyme determining the activity of the pyrimidine synthesis pathway in CRPC.
Although the specific metabolic pathways and final products may vary, multiple studies indicate that in tumor cells, the carbon and nitrogen metabolism of glutamine cooperate closely and are inseparable. More importantly, this co-operation ensures that when one metabolic pathway is obstructed, metabolic flux can be rerouted through another pathway to fulfill the biological function, ultimately achieving tumor cell's demand for glutamine. Wang et al. noted that under hypoxic conditions, tumor cells markedly enhance their use of glutamine carbon chains. Simultaneously, the nitrogen atoms of glutamine participate in the synthesis pathway of nucleotides, not as final products but are secreted extracellularly in the form of intermediate products such as lactate. This indicates that the carbon and nitrogen metabolic pathways of glutamine can mutually regulate under different environmental conditions to achieve the most suitable metabolic steady state [46]. Kodama et al. found that during tumor development, the carbon pathway of glutamine closely interacts with another nucleotide metabolic pathway - purine metabolism. The rate-limiting enzymes in both pathways, GLS1 and phosphoribosyl pyrophosphate amidotransferase (PPAT), maintain a dynamic equilibrium. When this balance is disrupted, the metabolic flux of glutamine shifts towards the more efficient pathway, ensuring continued utilization of glutamine by tumor cells [47]. Considering that the nitrogen metabolic pathway of glutamine likely compensates to maintain the consumption of glutamine by CRPC cells when the carbon metabolic pathway of glutamine is inhibited, theoretically, simultaneously inhibiting both the carbon and nitrogen metabolic pathways of glutamine can maximally restrict the utilization of glutamine by tumor cells. This approach can cut off the material source of energy and nutrients for tumor cells, thereby enhancing the therapeutic effect of CRPC.
Understanding of tumor cell metabolism, especially glutamine metabolism, is rapidly advancing. Significant progress has been made, yet many details remain elusive. Given the interplay of numerous interconnected metabolic pathways, tumor cells may trigger compensatory mechanisms to evade metabolic suppression. Given the highly intra-tumoral and inter-tumoral heterogeneity of prostate cancer, future research on metabolic changes between tumor cells and the tumor microenvironment (immune cells and stromal cells), as well as the interactions of different cellular components in different patients, will lead to more effective, precise, and personalized treatment approaches to assist a large number of patients in need.
It is noteworthy that the current research on the complexity and authenticity of metabolic characteristics primarily relies on cellular biology. However, it has the following limitations: (1). Culture conditions such as oxygen concentration in the laboratory-aided studies are vastly different from those in real tumors; (2). Geometric structure of cell culture is often conducted in two-dimensional layers, which cannot simulate the three-dimensional, dynamic heterogeneity of the tumor; (3). Composition and concentration of metabolites in cell culture media differ significantly from those in human blood or the tumor microenvironment; (4). Rapid growth and proliferation of cells in vitro also affect the translation of results. To overcome these technical obstacles, efforts are being made to develop new research models that closely mimic real-life conditions, thereby providing more reliable metabolic results. For example, organoid models are receiving widespread attention because they are generated from pluripotent or adult stem cells and possess the ability to simulate organ regeneration ex vivo. Animal models such as genetically engineered mouse models and patient-derived xenografts are also of great interest in cancer research and drug development. Since mice harbor almost all genes identical to humans, these models are suitable for metabolic experiments such as isotope tracing and metabolic imaging detection. In addition, because the human metabolic network encompasses thousands of enzymes, metabolites, and interactions, comprehensive analysis requires integration of various omics data.
None.
Ethical policy
All procedures performed in this study were in accordance with the ethical standards of the institutional and/or national research committee and with the 1964 Helsinki declaration and its later amendments or comparable ethical standards. Informed consent was obtained from all individual participants included in the study. Approval from institutional ethical committee was taken.
Availability of data and materials
All data generated or analysed during this study are included in this publication.
Author contributions
XG searched academic literature, wrote the draft manuscript, supervised the review writting progress and approved the final manuscript submission.
Competing interests
Authors report no conflict of interest.
Funding
None.
- Zanotelli MR, Zhang J, Reinhart-King CA: Mechanoresponsive metabolism in cancer cell migration and metastasis. Cell Metab 2021, 33(7): 1307-1321.
- Fernie AR, Carrari F, Sweetlove LJ: Respiratory metabolism: glycolysis, the TCA cycle and mitochondrial electron transport. Curr Opin Plant Biol 2004, 7(3): 254-261.
- Vander Heiden MG, Cantley LC, Thompson CB: Understanding the Warburg effect: the metabolic requirements of cell proliferation. Science 2009, 324(5930): 1029-1033.
- Malumbres M, Barbacid M: RAS oncogenes: the first 30 years. Nat Rev Cancer 2003, 3(9): 708-717.
- Newsholme EA, Crabtree B, Ardawi MS: The role of high rates of glycolysis and glutamine utilization in rapidly dividing cells. Biosci Rep 1985, 5(5): 393-400.
- Wasim S, Lee SY, Kim J: Complexities of Prostate Cancer. Int J Mol Sci 2022, 23(22): 14257-1477.
- Chen W, Zheng R, Baade PD, Zhang S, Zeng H, Bray F, Jemal A, Yu XQ, He J: Cancer statistics in China, 2015. CA Cancer J Clin 2016, 66(2): 115-132.
- Teo MY, Rathkopf DE, Kantoff P: Treatment of Advanced Prostate Cancer. Annu Rev Med 2019, 70: 479-499.
- Flavin R, Zadra G, Loda M: Metabolic alterations and targeted therapies in prostate cancer. J Pathol 2011, 223(2): 283-294.
- Xu L, Ma E, Zeng T, Zhao R, Tao Y, Chen X, Groth J, Liang C, Hu H, Huang J: ATM deficiency promotes progression of CRPC by enhancing Warburg effect. Endocr Relat Cancer 2019, 26(1): 59-71.
- Xu L, Yin Y, Li Y, Chen X, Chang Y, Zhang H, Liu J, Beasley J, McCaw P, Zhang H: A glutaminase isoform switch drives therapeutic resistance and disease progression of prostate cancer. Proc Natl Acad Sci U S A 2021, 118(13): e2012748118.
- Li W, Cohen A, Sun Y, Squires J, Braas D, Graeber TG, Du L, Li G, Li Z, Xu X: The Role of CD44 in Glucose Metabolism in Prostatic Small Cell Neuroendocrine Carcinoma. Mol Cancer Res 2016, 14(4): 344-353.
- Yao M, Rogers L, Suchowerska N, Choe D, Al-Dabbas MA, Narula RS, Lyons JG, Sved P, Li Z, Dong Q: Sensitization of prostate cancer to radiation therapy: Molecules and pathways to target. Radiother Oncol 2018, 128(2): 283-300.
- Roth E: Nonnutritive effects of glutamine. J Nutr 2008, 138(10): 2025S-2031S.
- Newsholme EA, Parry-Billings M: Properties of glutamine release from muscle and its importance for the immune system. JPEN J Parenter Enteral Nutr 1990, 14(4 Suppl): 63S-67S.
- Wernerman J: Clinical use of glutamine supplementation. J Nutr 2008, 138(10): 2040S-2044S.
- Berg A, Norberg A, Martling CR, Gamrin L, Rooyackers O, Wernerman J: Glutamine kinetics during intravenous glutamine supplementation in ICU patients on continuous renal replacement therapy. Intensive Care Med 2007, 33(4): 660-666.
- Labow BI, Souba WW, Abcouwer SF: Mechanisms governing the expression of the enzymes of glutamine metabolism--glutaminase and glutamine synthetase. J Nutr 2001, 131(9 Suppl): 2467S-2477S.
- Cooney G, Curi R, Mitchelson A, Newsholme P, Simpson M, Newsholme EA: Activities of some key enzymes of carbohydrate, ketone body, adenosine and glutamine metabolism in liver, and brown and white adipose tissues of the rat. Biochem Biophys Res Commun 1986, 138(2): 687-692.
- Tan HWS, Sim AYL, Long YC: Glutamine metabolism regulates autophagy-dependent mTORC1 reactivation during amino acid starvation. Nat Commun 2017, 8(1): 338-348.
- Newsholme P: Why is L-glutamine metabolism important to cells of the immune system in health, postinjury, surgery or infection? J Nutr 2001, 131(9 Suppl): 2515S-2524S.
- Ardawi MS: Glutamine metabolism in the lungs of glucocorticoid-treated rats. Clin Sci (Lond) 1991, 81(1): 37-42.
- Parry-Billings M, Dimitriadis GD, Leighton B, Bond J, Bevan SJ, Opara E, Newsholme EA: Effects of hyperthyroidism and hypothyroidism on glutamine metabolism by skeletal muscle of the rat. Biochem J 1990, 272(2): 319-322.
- Parry-Billings M, Dimitriadis GD, Leighton B, Dunger DB, Newsholme EA: The effects of growth hormone administration in vivo on skeletal muscle glutamine metabolism of the rat. Horm Metab Res 1993, 25(6): 292-293.
- Cruzat VF, Keane KN, Scheinpflug AL, Cordeiro R, Soares MJ, Newsholme P: Alanyl-glutamine improves pancreatic β-cell function following ex vivo inflammatory challenge. J Endocrinol 2015, 224(3): 261-271.
- Krebs HA: Metabolism of amino-acids: The synthesis of glutamine from glutamic acid and ammonia, and the enzymic hydrolysis of glutamine in animal tissues. Biochem J 1935, 29(8): 1951-1969.
- Neu J, Shenoy V, Chakrabarti R: Glutamine nutrition and metabolism: where do we go from here ? FASEB J 1996, 10(8): 829-837.
- Curi R, Newsholme P, Marzuca-Nassr GN, Takahashi HK, Hirabara SM, Cruzat V, Krause M, de Bittencourt PI Jr: Regulatory principles in metabolism-then and now. Biochem J 2016, 473(13): 1845-1857.
- Holeček M: Branched-chain amino acids in health and disease: metabolism, alterations in blood plasma, and as supplements. Nutr Metab (Lond) 2018, 15(1): 33-46.
- Cruzat VF, Krause M, Newsholme P: Amino acid supplementation and impact on immune function in the context of exercise. J Int Soc Sports Nutr 2014, 11(1): 61-74.
- Holeček M: Branched-chain amino acids in health and disease: metabolism, alterations in blood plasma, and as supplements. Nutr Metab (Lond) 2018, 15 (1): 33-35.
- Altman BJ, Stine ZE, Dang CV: From Krebs to clinic: glutamine metabolism to cancer therapy. Nat Rev Cancer 2016, 16(11): 619-634.
- Cruzat VF, Pantaleão LC, Donato J Jr, de Bittencourt PI Jr, Tirapegui J: Oral supplementations with free and dipeptide forms of L-glutamine in endotoxemic mice: effects on muscle glutamine-glutathione axis and heat shock proteins. J Nutr Biochem 2014, 25(3): 345-352.
- Karinch AM, Pan M, Lin CM, Strange R, Souba WW: Glutamine metabolism in sepsis and infection. J Nutr 2001, 131(9 Suppl): 2535S-2541S.
- Fläring UB, Rooyackers OE, Wernerman J, Hammarqvist F: Glutamine attenuates post-traumatic glutathione depletion in human muscle. Clin Sci (Lond) 2003, 104(3): 275-282.
- Rodas PC, Rooyackers O, Hebert C, Norberg Å, Wernerman J: Glutamine and glutathione at ICU admission in relation to outcome. Clin Sci (Lond) 2012, 122(12): 591-597.
- Leite JS, Raizel R, Hypólito TM, Rosa TD, Cruzat VF, Tirapegui J: l-glutamine and l-alanine supplementation increase glutamine-glutathione axis and muscle HSP-27 in rats trained using a progressive high-intensity resistance exercise. Appl Physiol Nutr Metab 2016, 41(8): 842-849.
- Cruzat VF, Krause M, Newsholme P: Amino acid supplementation and impact on immune function in the context of exercise. J Int Soc Sports Nutr 2014, 11(1): 61-74.
- Curi R, Lagranha CJ, Doi SQ, Sellitti DF, Procopio J, Pithon-Curi TC: Glutamine-dependent changes in gene expression and protein activity. Cell Biochem Funct 2005, 23(2): 77-84.
- Djoko KY, Phan MD, Peters KM, Walker MJ, Schembri MA, McEwan AG: Interplay between tolerance mechanisms to copper and acid stress in Escherichia coli. Proc Natl Acad Sci U S A 2017, 114(26): 6818-6823.
- Strmiska V, Michalek P, Eckschlager T, Stiborova M, Adam V, Krizkova S, Heger Z: Prostate cancer-specific hallmarks of amino acids metabolism: Towards a paradigm of precision medicine. Biochim Biophys Acta Rev Cancer 2019, 1871(2): 248-258.
- Yang WH, Qiu Y, Stamatatos O, Janowitz T, Lukey MJ: Enhancing the Efficacy of Glutamine Metabolism Inhibitors in Cancer Therapy. Trends Cancer 2021, 7(8): 790-804.
- Xu L, Zhao B, Butler W, Xu H, Song N, Chen X, Hauck JS, Gao X, Zhang H, Groth J: Targeting glutamine metabolism network for the treatment of therapy-resistant prostate cancer. Oncogene 2022, 41(8): 1140-1154.
- Bott AJ, Maimouni S, Zong WX: The Pleiotropic Effects of Glutamine Metabolism in Cancer. Cancers (Basel) 2019, 11(6): 770-786.
- Wang Y, Bai C, Ruan Y, Liu M, Chu Q, Qiu L, Yang C, Li B: Coordinative metabolism of glutamine carbon and nitrogen in proliferating cancer cells under hypoxia. Nat Commun 2019, 10(1): 201-215.
- Kodama M, Oshikawa K, Shimizu H, Yoshioka S, Takahashi M, Izumi Y, Bamba T, Tateishi C, Tomonaga T, Matsumoto M: A shift in glutamine nitrogen metabolism contributes to the malignant progression of cancer. Nat Commun 2020, 11(1): 1320-1336.
- Yin Y, Xu L, Chang Y, Zeng T, Chen X, Wang A, Groth J, Foo WC, Liang C, Hu H: N-Myc promotes therapeutic resistance development of neuroendocrine prostate cancer by differentially regulating miR-421/ATM pathway. Mol Cancer 2019, 18(1): 11-24.
- Xu L, Chen J, Liu W, Liang C, Hu H, Huang J: Targeting androgen receptor-independent pathways in therapy-resistant prostate cancer. Asian J Urol 2019, 6(1): 91-98.
- Tan MH, Li J, Xu HE, Melcher K, Yong EL: Androgen receptor: structure, role in prostate cancer and drug discovery. Acta Pharmacol Sin 2015, 36(1): 3-23.
- Aurilio G, Cimadamore A, Mazzucchelli R, Lopez-Beltran A, Verri E, Scarpelli M, Massari F, Cheng L, Santoni M, Montironi R: Androgen Receptor Signaling Pathway in Prostate Cancer: From Genetics to Clinical Applications. Cells 2020, 9(12): 2653-2667.
- Ather MH, Abbas F, Faruqui N, Israr M, Pervez S: Correlation of three immunohistochemically detected markers of neuroendocrine differentiation with clinical predictors of disease progression in prostate cancer. BMC Urol 2008, 8(1): 21-26.
- Choi YK, Park KG: Targeting Glutamine Metabolism for Cancer Treatment. Biomol Ther (Seoul) 2018, 26(1): 19-28.
- Choi SYC, Ettinger SL, Lin D, Xue H, Ci X, Nabavi N, Bell RH, Mo F, Gout PW, Fleshner NE: Targeting MCT4 to reduce lactic acid secretion and glycolysis for treatment of neuroendocrine prostate cancer. Cancer Med 2018, 7(7): 3385-3392.
- Li Y, He Y, Butler W, Xu L, Chang Y, Lei K, Zhang H, Zhou Y, Gao AC, Zhang Q: Targeting cellular heterogeneity with CXCR2 blockade for the treatment of therapy-resistant prostate cancer. Sci Transl Med 2019, 11(521): eaax0428.
Annals of urologic oncology
p-ISSN: 2617-7765, e-ISSN: 2617-7773
Copyright © Ann Urol Oncol. This work is licensed under a Creative Commons Attribution-NonCommercial-No Derivatives 4.0 International (CC BY-NC-ND 4.0) License.