Review Article | Open Access
Advances in Prostate Cancer Immunotherapy: Current Options and Emerging Novel Approaches
Nasser S Alanazi1, Mohammed H Alrafiah1
1Department of Medical Laboratory, College of Applied Medical Sciences, Prince Sattam bin Abdulaziz University, Al-Kharj 11942, Saudi Arabia.
Correspondence: Mohammed H Alrafiah (Department of Medical Laboratory, College of Applied Medical Sciences, Prince Sattam bin Abdulaziz University, Al-Kharj 11942, Saudi Arabia; Email: iimoh323@gmail.com).
Annals of Urologic Oncology 2024, 7: 21. https://doi.org/10.32948/auo.2024.10.20
Received: 10 Oct 2024 | Accepted: 22 Oct 2024 | Published online: 26 Oct 2024
Key words clinical trials, gene therapy, nanotechnology, prostate cancer, immunotherapy
The pathogenesis of PCa involves a gradual and ongoing progression characterized by the development of small tumors that slowly transform into distinct clonal entities, each with varying clinical outcomes [16]. Research has indicated that chronic inflammation is commonly observed in the prostates of older men and is linked to a heightened risk of developing PCa [17]. However, the precise mechanisms behind chronic prostate inflammation and its clinical significance in the progression of PCa remain uncertain. In spite of this, clinical data indicates that persistent inflammation could be a risk factor for the advancement of the disease and poor clinical results [18].
Innovative strategies are critical for advancing immunotherapies in prostate cancer (PCa). The success of Sipuleucel-T has shown that T-cell-based therapies can be clinically effective, opening the door to further progress in this field. One of the most promising new strategies is the use of bispecific T-cell engagers, particularly in treating non-inflamed tumors like PCa. These agents work by targeting cancer-specific epitopes, such as prostate-specific membrane antigen (PSMA), and linking them to a portion of the T-cell receptor. This redirection of T-cells to the tumor environment stimulates an immune response by activating T-cells and recruiting them to the tumor site. This review will explore immunotherapy strategies for PCa that have the potential to revolutionize the management of both localized and advanced disease stages [19].
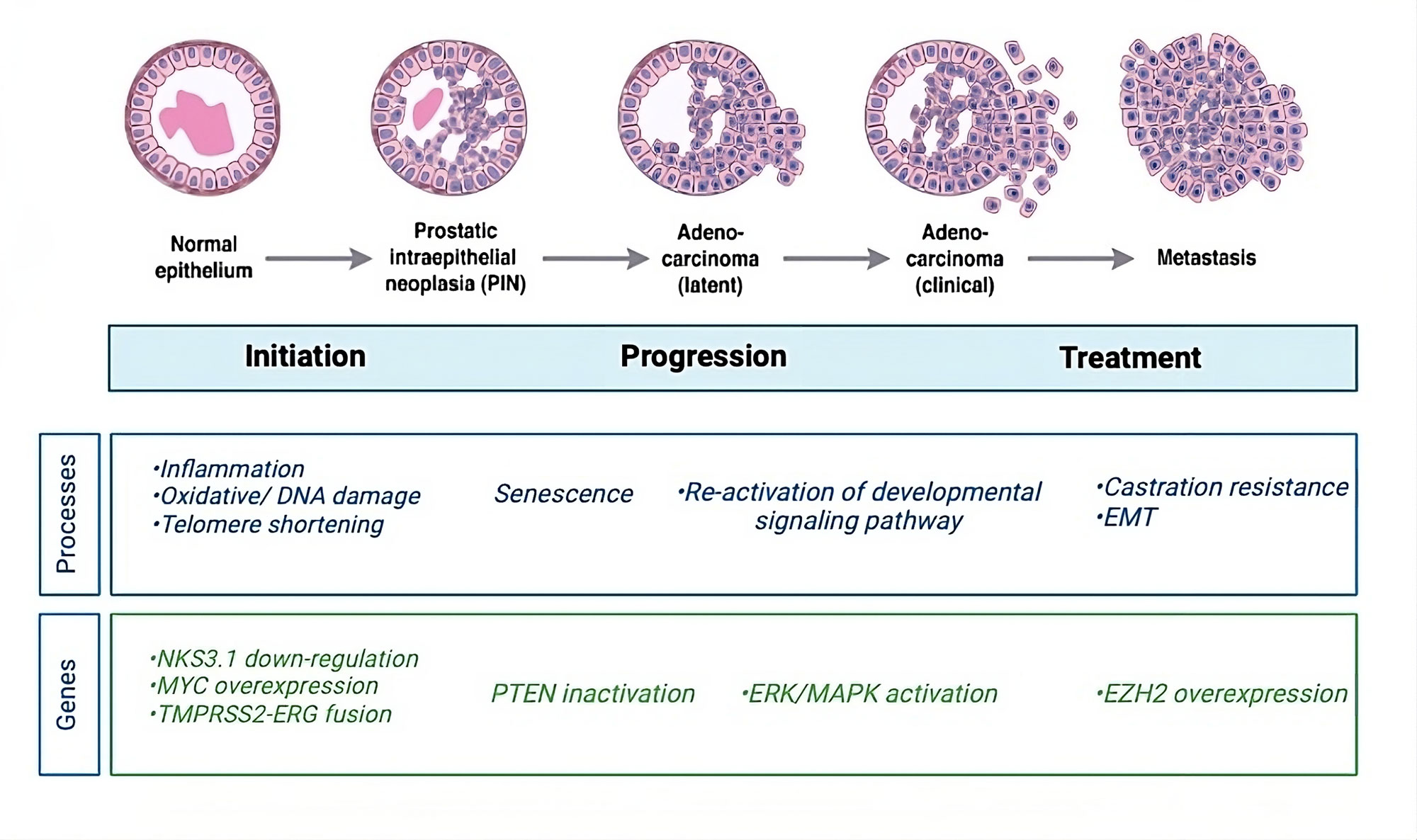
Patients with metastatic PCa exhibit disrupted cellular immunity and a tumor microenvironment characterized by heightened immunosuppressive features. The compromised immune response in these patients is marked by reduced natural killer (NK) cell activity and renewal, as well as diminished expression of CD3 on NK and T cells, potentially leading to a decrease in T cell receptors and NK cell-activating receptors [27]. Additionally, myeloid-derived suppressor cells and regulatory T cells are more prevalent in the tumor microenvironment and bloodstream of patients with mCRPC [28], and they also often have fewer total T cells [29]. The delayed evolution of PCa may also be a contributing factor to immunotherapy resistance and tolerance [30]. De novo resistance to immunotherapy may also arise as a result of the low mutational burden seen in PCa patients (Figure 2) [31]. However, this perspective is still debated, as recent genomic analyses have suggested that PCa patients may actually exhibit a higher tumor mutation burden compared to those with renal cell carcinoma [32]. Additional research is needed to clarify the specific pathological mechanisms contributing to the resistance of PCa patients to immunotherapy, ultimately aiming to develop effective immunotherapeutic strategies for metastatic PCa.
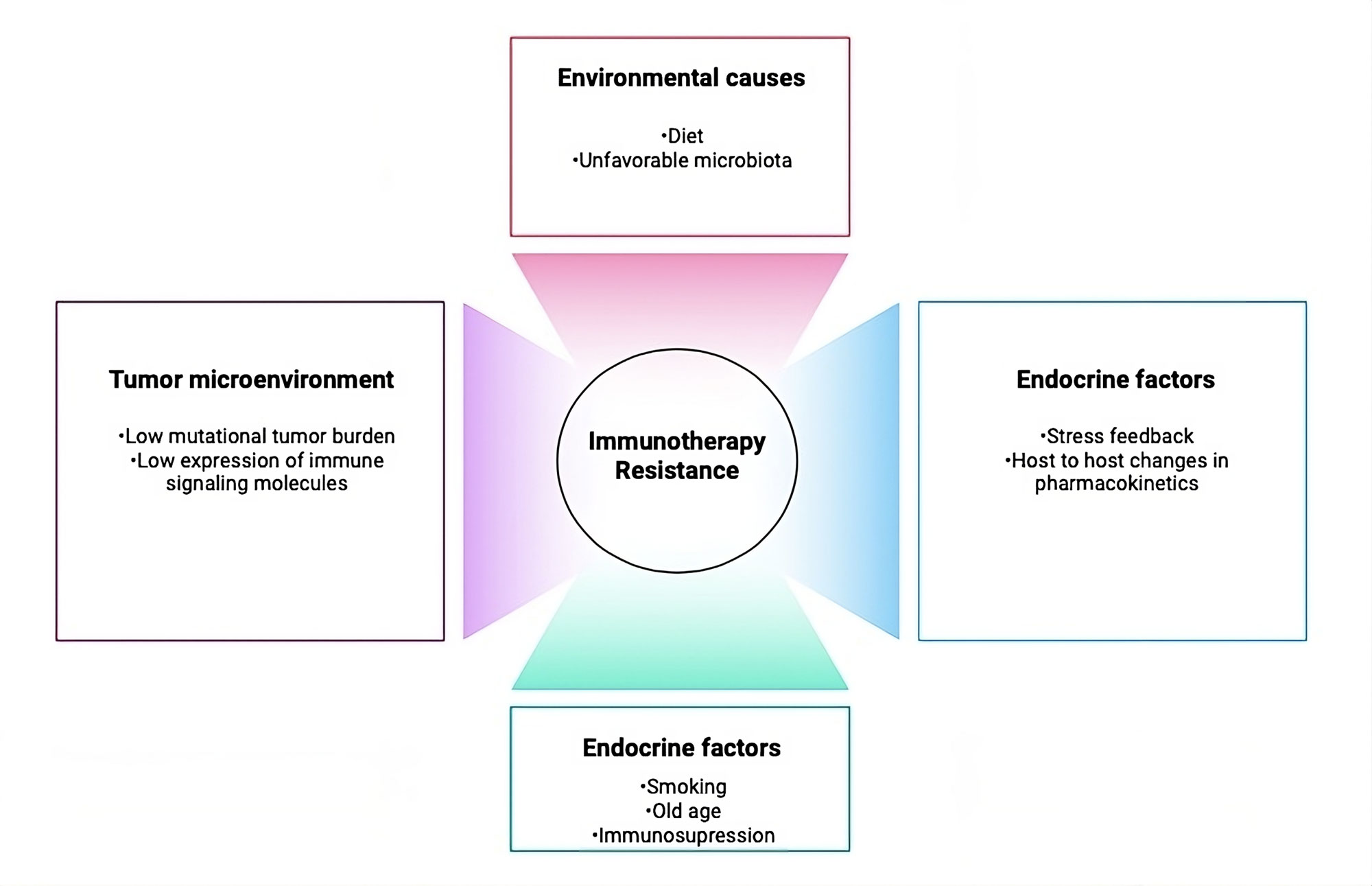
DNA vaccines have primarily been studied in animal models as a potential cancer treatment. Their application in humans remains contentious due to the associated risks versus benefits [35]. These vaccines provide a novel approach compared to traditional anti-tumor vaccinations, offering advantages such as ease of administration and the absence of infectious agents. DNA vaccines for PCa are currently being studied in a number of Phase 1 clinical trials. One such experiment, NCT02411786 by Madison Vaccines Inc., targets androgen receptors using the pTVG-AR and MVI-118 designs [36]. Furthermore, a Phase I/II trial involving patients with biochemically relapsed PCa has assessed Inovio Pharmaceuticals' dual-antigen DNA vaccine INO-5150, which combines elements of PSMA and PSA [37].
In order to elicit an immunological response in the body, the commercial vaccine PROSTVAC uses a recombinant strain of vaccinia in conjunction with transgenes, boosts from the fowlpox vector, and co-stimulatory molecules [38]. PSA-specific T-cell counts have increased in patients receiving PROSTVAC treatment [39]. PROSTVAC may be beneficial for patients with mCRPC, according to two Phase II studies. In one research, PROSTVAC or a placebo was randomly assigned to 125 mCRPC patients with a Gleason score of ≤7 [40]. Patients receiving the placebo had a median survival rate of 16.3 months, while those treated with PROSTVAC had a median survival rate of 24.4 months [41]. In an attempt to explore this theory further, a recent Phase III trial was carried out, however it failed to show any appreciable therapeutic improvement [39]. Despite being well-tolerated and able to stimulate the immune system, the vaccine offered very little in the way of survival benefits [42].
Using genetically engineered entire prostate cancer cells, known as GVAX, the tumor cells can act as the source of antigen for immunotherapy since they release the immune-stimulatory cytokine granulocyte-macrophage colony-stimulating factor [43]. GVAX has demonstrated safety and effectiveness as a potent cytokine, producing a significant immune response that is dose-dependent. Patients treated with GVAX typically experienced only mild side effects, such as flu-like symptoms and fever. Nevertheless, due to several unsuccessful Phase 3 trials involving this vaccine, further research has largely been discontinued [44].
Chimeric antigen receptors (CAR)-modified epithelial cell adhesion molecule (EpCAM)-targeted T-cells have shown promise in a range of cancer immunotherapies utilizing this stem cell antigen. Studies on low-expression human prostate cancer cells have shown that these cells are highly effective at preventing tumor growth in both vitro and in vivo settings [47]. Additionally, it appears that the Natural Killer Group 2D (NKG2D) receptor is a viable target for CAR T-cell treatment. In conjunction with the IL-7 gene, it has demonstrated efficacy in the management of PCa [48].
To achieve more precise targeting of prostate cancer, CAR T-cells have frequently been developed against PSMA. In the first-in-human Phase 1 trial of PSMA-targeting CAR T-cells armed with a dominant-negative TGF-β receptor (NCT03089203) in patients with castration-resistant prostate cancer (CRPC), 5 out of 13 patients experienced cytokine release syndrome (CRS) of grade 2 or higher, and 4 patients showed decreases of 30% or more in PSA levels. Notably, one patient exhibited a greater than 98% reduction in PSA, along with evidence of significant clonal expansion of CAR T-cells. However, this patient subsequently developed enterococcal sepsis 30 days post-infusion, resulting in multi-organ dysfunction and death [49]. Three of the nine patients in a different ongoing clinical trial with PSMA-targeting CAR for metastatic CRPC saw improvements in PSMA positron-emission tomography imaging and a drop in PSA levels of more than 50% [49]. One patient achieved total clearance of detectable illness for almost five months, while three others had CRS of grades 1-2 [50]. Even though these studies' findings are encouraging, CAR T-cell treatment still faces several obstacles. Overcoming the immunosuppressive tumor microenvironment (TME), which is full of growth factors and immunosuppressive cytokines, as well as possible toxicities related to the therapy are major constraints.
Another approach to ACT is Tumor-Infiltrating Lymphocyte (TIL) therapy, which focuses on analyzing specific lymphocytes found around tumors. In this method, T-cells that are most effective at recognizing malignant tumor cells are isolated, treated, and stimulated to proliferate in the tumor environment. However, incorporating TIL-based immunotherapy into PCa treatment poses challenges due to the disease's T-cell exclusive nature [51]. This difficulty can be attributed to the relatively low genomic complexity of prostate cancer cells compared to other cancers [52]. Recent studies have shown that it is feasible to extract functional and tumor-reactive TILs from prostate cancer. In one study, researchers successfully extracted and expanded twenty-eight prostate-TIL cultures in the laboratory, achieving an expansion frequency of approximately 50% across all samples. Following expansion, these TILs exhibited clear expression of chemokine receptors. Further exploration of this therapeutic approach could lead to new treatment modalities for patients [53].
A significant challenge in both CAR T-cell therapy and TIL therapy is maintaining long-term proliferation in immunosuppressive environments. Consequently, there is a growing emphasis on research aimed at enhancing the survival rates of CAR T-cells, particularly through the integration of TILs expressing 4-1BB and CD137 receptors [54]. The incorporation of such therapies into patient care represents an effective treatment strategy with fewer side effects compared to other cancer treatment modalities.
Bispecific T-cell engagers (BiTEs) have already been successfully implemented in treating various malignancies. The first BiTE therapy to receive approval was Blinatumumab, a bispecific monoclonal antibody construct designed to enable CD3-positive T-cells to recognize and target CD19-positive B-cells. Blinatumumab is indicated for patients with refractory or relapsed precursor B-cell acute lymphoblastic leukemia (B-ALL). In comparison to chemotherapy, blinatumumab demonstrated a survival advantage for patients with pretreated B-ALL, with a median overall survival (OS) of 7.7 months in the blinatumumab group versus 4.0 months in the chemotherapy group (HR = 0.71; 95% CI 0.55 to 0.93; p = 0.01) [56].
Given the encouraging results in treating hematological diseases, researchers are currently exploring the potential of bispecific T-cell engagers (BiTEs) for solid tumors. A Phase I trial was conducted to evaluate the tolerability and efficacy of pasotuximab (PSMA Bite) in patients with mCRPC. This study involved 68 participants divided into two groups receiving either subcutaneous (s.c.) or intravenous (i.v.) administration. All patients had undergone at least one prior taxane treatment and were refractory to abiraterone acetate (AAP) or enzalutamide. The trial aimed to assess the maximum tolerated dose in both groups while monitoring PSA responses. However, in the subcutaneous cohort, all patients developed anti-drug antibodies, resulting in the early termination of this treatment group [57].
In the Phase I study of pasotuximab (PSMA Bite), patients in the subcutaneous (s.c.) cohort showed a PSA decline of −24.7%. In the intravenous (i.v.) cohort, the median best PSA reductions were −22.0%, −37.7%, and −54.9% for the 20, 40, and 80 µg/d dose groups, respectively. One patient in the i.v. group experienced a PSA reduction of less than 50% for 50 weeks and stable disease for 337 days, while another patient had nearly complete regression of lymph node and bone metastases, as seen on PSMA-PET CT. Long-term responders in the i.v. cohort showed PSA progression after 11–17 months, suggesting dose-dependent activity of the treatment. The most common adverse events (AEs) were fever, seen in 81% of the s.c. cohort and 94% of the i.v. cohort, injection site reactions in the s.c. group (77%), chills (23% in s.c. and 69% in i.v. groups), and fatigue (36% in s.c. and 31% in i.v. groups). Treatment-emergent AEs were frequent, with anemia occurring in 39% of the s.c. group, while decreased lymphocyte counts (44%) and infections (31%) were common in the i.v. cohortition to PSMA [57], other targets for bispecific T-cell engagers are under investigation, including Glypican-1, ADAM 17P-1 [58-60]. Overall, bispecific T-cell engagers represent a promising new therapeutic option, showing early signs of clinical efficacy. While further studies are necessary to fully establish their safety and effectiveness, early clinical trials are encouraging.
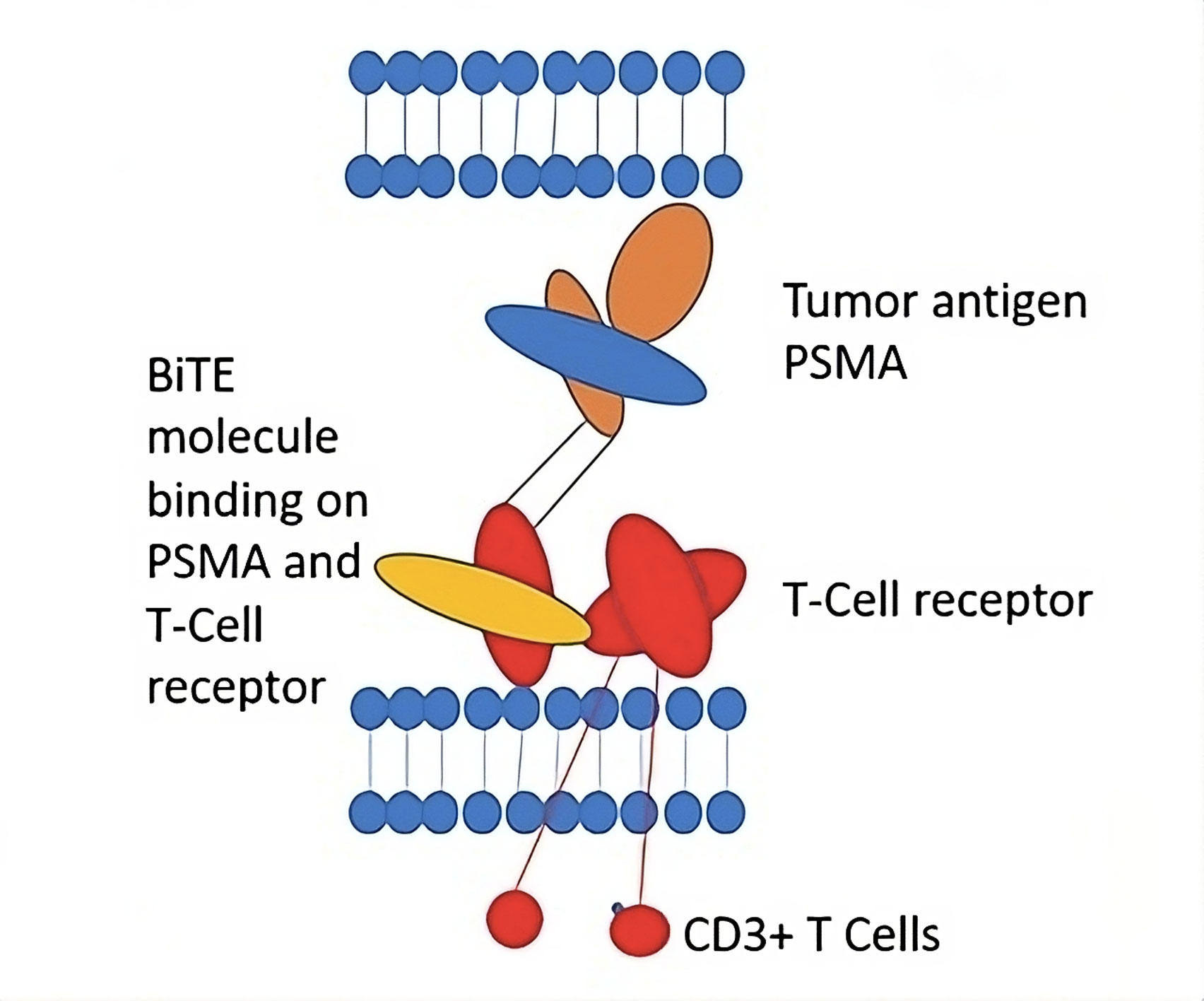
Clinical trials for PCa have extensively investigated the use of magnetic nanoparticles as a contemporary method of tumor heating. In one study, magnetic nanoparticle thermotherapy was investigated, either in isolation or in conjunction with permanent seed brachytherapy. The viability and tolerability of this approach were evaluated using the first prototype of an alternating magnetic field applicator. The results showed that, even at modest magnetic field strengths of 4-5 kA/m, magnetic nanoparticle thermotherapy may cause hyperthermia and raise the prostate's temperature to thermoablative levels [78]. With advances in molecular techniques, a new generation of large-scale, population-based studies has been initiated to evaluate both the individual and combined effects of these treatments [79].
None.
Ethical policy
Non applicable.
Availability of data and materials
All data generated or analysed during this study are included in this publication.
Author contributions
NSA searched academic literature, wrote the draft manuscript; MHA supervised the review writting progress and approved the final manuscript submission.
Competing interests
Authors report no conflict of interest.
Funding
None.
- Sung H, Ferlay J, Siegel RL, Laversanne M, Soerjomataram I, Jemal A, Bray F: Global cancer statistics 2020: GLOBOCAN estimates of incidence and mortality worldwide for 36 cancers in 185 countries. CA Cancer J Clin 2021, 71(3): 209-249.
- Kouspou MM, Fong JE, Brew N, Hsiao ST, Davidson SL, Choyke PL, Crispino T, Jain S, Jenster GW, Knudsen BS et al: The Movember Prostate Cancer Landscape Analysis: an assessment of unmet research needs. Nat Rev Urol 2020, 17(9): 499-512.
- Løvf M, Zhao S, Axcrona U, Johannessen B, Bakken AC, Carm KT, Hoff AM, Myklebost O, Meza-Zepeda LA, Lie AK et al: Multifocal primary prostate cancer exhibits high degree of genomic heterogeneity. Eur Urol 2019, 75(3): 498-505.
- Andreoiu M, Cheng L: Multifocal prostate cancer: biologic, prognostic, and therapeutic implications. Human Pathol 2010, 41(6): 781-793.
- Wei L, Wang J, Lampert E, Schlanger S, DePriest AD, Hu Q, Gomez EC, Murakam M, Glenn ST, Conroy J et al: Intratumoral and intertumoral genomic heterogeneity of multifocal localized prostate cancer impacts molecular classifications and genomic prognosticators. Eur Urol 2017 71(2): 183-192.
- Lindberg J, Klevebring D, Liu W, Neiman M, Xu J, Wiklund P, Wiklund F, Mills IG, Egevad L, Grönberg H et al: Exome sequencing of prostate cancer supports the hypothesis of independent tumour origins. Eur rol 2013, 63(2): 347-353.
- Su F, Zhang W, Zhang D, Zhang Y, Pang C, Huang Y, Wang M, Cui L, He L, Zhang J et al: Spatial intratumor genomic heterogeneity within localized prostate cancer revealed by single-nucleus sequencing. Eur Urol 2018, 74(5): 551-559.
- Groden J, Thliveris A, Samowitz W, Carlson M, Gelbert L, Albertsen H, Joslyn G, Stevens J, Spirio L, Robertson M et al: Identification and characterization of the familial adenomatous polyposis coli gene. Cell 1991, 66(3): 589-600.
- Shen MM, Abate-Shen C: Molecular genetics of prostate cancer: new prospects for old challenges. Genes Dev 2010, 24(18): 1967-2000.
- Amirrad F, Pytak PA, SadeghianiPelar N, Nguyen J, Cauble EL, Jones AC, Bisoffi M: Prostate field cancerization and exosomes: Association between CD9, early growth response 1 and fatty acid synthase. Int J Oncol 2020, 56(4): 957-968.
- Tretiakova MS, Wei W, Boyer HD, Newcomb LF, Hawley S, Auman H, Vakar-Lopez F, McKenney JK, Fazli L, Simko J et al: Prognostic value of Ki67 in localized prostate carcinoma: a multi-institutional study of > 1000 prostatectomies. Prostate Cancer Prostatic Dis 2016, 19(3): 264-270.
- Catalona WJ, Smith DS, Ratliff TL, Basler JW: Detection of organ-confined prostate cancer is increased through prostate-specific antigen-bases screening. JAMA 1993, 270 (8): 948-954.
- Hole KH, Axcrona K, Lie AK, Vlatkovic L, Geier OM, Brennhovd B, Knutstad K, Olsen DR, Seierstad T: Routine pelvic MRI using phased-array coil for detection of extraprostatic tumour extension: accuracy and clinical significance. Eur Radiol 2013, 23(4): 1158-1166.
- Baco E, Ukimura O, Rud E, Vlatkovic L, Svindland A, Aron M, Palmer S, Matsugasumi T, Marien A, Bernhard JC: Magnetic resonance imaging-transectal ultrasound image-fusion biopsies accurately characterize the index tumor: correlation with step-sectioned radical prostatectomy specimens in 135 patients. Eur Urol 2015, 67(4):787-794.
- Donaldson IA, Alonzi R, Barratt D, Barret E, Berge V, Bott S, Bottomley D, Eggener S, Ehdaie B, Emberton M et al: Focal therapy: patients, interventions, and outcomes-a report from a consensus meeting. Eur Urol 2015, 67(4): 771-777.
- Abeshouse A, Ahn J, Akbani R, Ally A, Amin S, Andry CD, Annala M, Aprikian A, Armenia J, Arora A et al: The molecular taxonomy of primary prostate cancer. Cell 2015, 163(4): 1011-1025.
- Platz EA, Kulac I, Barber JR, Drake CG, Joshu CE, Nelson WG, Lucia MS, Klein EA, Lippman SM, Parnes HL et al: A prospective study of chronic inflammation in benign prostate tissue and risk of prostate cancer: linked PCPT and SELECT cohorts. Cancer Epidemiol Biomarkers Prev 2017, 26(10): 1549-1557.
- Iczkowski KA, Torkko KC, Wilson RS, Lucia MS, Bostwick DG: Prostatic atrophy: its spatial proximity to carcinoma and intraepithelial neoplasia based on annotation of digital slides. Hum Pathol 2014, 45(1): 54-58.
- Kim TJ, Koo KC: Current status and future perspectives of checkpoint inhibitor immunotherapy for prostate cancer: a comprehensive review. Int J Mol Sci 2020, 21(15): 5484.
- Graff JN, Liang LW, Kim J, Stenzl A: KEYNOTE-641: a Phase III study of pembrolizumab plus enzalutamide for metastatic castration-resistant prostate cancer. Future Oncol 2021, 17(23): 3017-3026.
- Petrylak DP, Ratta R, Gafanov R, Facchini G, Piulats JM, Kramer G, Flaig TW, Chandana SR, Li B, Burgents J et al: KEYNOTE-921: Phase III study of pembrolizumab plus docetaxel for metastatic castration-resistant prostate cancer. Future Oncol 2021, 17(25): 3291-3299.
- Gratzke C, Niu C, Poehlein C, Burgents J: 346 KEYNOTE-991: Phase 3 study of pembrolizumab plus enzalutamide and androgen deprivation therapy (ADT) for patients with metastatic hormone-sensitive prostate cancer (mHSPC). J Immunother Cancer 2020, 8: A371.
- Joshua AM, Gurney H, Retz M, Tafreshi A, Fong PC, Shore ND, Romano E, Augustin M, Piulats JM, Berry WR et al: 217O Pembrolizumab (pembro) combination therapies in patients with metastatic castration-resistant prostate cancer (mCRPC): Cohorts AC of the phase Ib/II KEYNOTE-365 study. Ann Oncol 2020, 31: S1325.
- Kumar V, Patel S, Tcyganov E, Gabrilovich DI: The nature of myeloid-derived suppressor cells in the tumor microenvironment. Trends Immunol 2016, 37(3): 208-220.
- Chabanon RM, Pedrero M, Lefebvre C, Marabelle A, Soria JC, Postel-Vinay S: Mutational landscape and sensitivity to immune checkpoint blockers. Clin Canc Res 2016, 22(17): 4309-4321.
- McGranahan N, Furness AJ, Rosenthal R, Ramskov S, Lyngaa R, Saini SK, Jamal-Hanjani M, Wilson GA, Birkbak NJ, Hiley CT et al: Clonal neoantigens elicit T cell immunoreactivity and sensitivity to immune checkpoint blockade. Science 2016, 351(6280): 1463-1469.
- Liu G, Lu S, Wang X, Page ST, Higano CS, Plymate SR, Greenberg NM, Sun S, Li Z, Wu JD et al: Perturbation of NK cell peripheral homeostasis accelerates prostate carcinoma metastasis. J Clin Invest 2013, 123(10): 4410-4422.
- Pal SK, Moreira D, Won H, White SW, Duttagupta P, Lucia M, Jones J, Hsu J, Kortylewski M et al: Reduced T-cell numbers and elevated levels of immunomodulatory cytokines in metastatic prostate cancer patients de novo resistant to abiraterone and/or enzalutamide therapy. Intl J Mol Sci 2019, 20(8): 1831.
- Chi N, Tan Z, Ma K, Bao L, Yun Z: Increased circulating myeloid-derived suppressor cells correlate with cancer stages, interleukin-8 and-6 in prostate cancer. Intl J Clin Exp Med 2014, 7(10): 3181-3192
- Zlotta AR, Egawa S, Pushkar D, Govorov A, Kimura T, Kido M, Takahashi H, Kuk C, Kovylina M, Aldaoud N et al: Prevalence of prostate cancer on autopsy: cross-sectional study on unscreened Caucasian and Asian men. J Natl Cancer Inst 2013, 105(14): 1050-8.
- Maleki Vareki S: High and low mutational burden tumors versus immunologically hot and cold tumors and response to immune checkpoint inhibitors. J Immunother Cancer 2018, 6(1): 157.
- Chen YP, Zhang Y, Lv JW, Li YQ, Wang YQ, He QM, Yang XJ, Sun Y, Mao YP, Yun JP et al: Genomic analysis of tumor microenvironment immune types across 14 solid cancer types: immunotherapeutic implications. Theranostics 2017, 7(14): 3585-3594.
- Cheever MA, Higano CS: PROVENGE (Sipuleucel-T) in prostate cancer: the first FDA-approved therapeutic cancer vaccine. Clin Cancer Res 2011, 17(11): 3520-3526.
- Kantoff PW, Higano CS, Shore ND, Berger ER, Small EJ, Penson DF, Redfern CH, Ferrari AC, Dreicer R et al: Sipuleucel-T immunotherapy for castration-resistant prostate cancer. New Engl J Med 2010, 363(5): 411-422.
- Kobiyama K, Jounai N, Aoshi T, Tozuka M, Takeshita F, Coban C, Ishii KJ: Innate immune signaling by, and genetic adjuvants for DNA vaccination. Vaccines 2013, 1(3): 278-292.
- Kyriakopoulos CE, Eickhoff JC, Ferrari AC, Schweizer MT, Wargowski E, Olson BM, McNeel DG: Multicenter phase I trial of a DNA vaccine encoding the androgen receptor ligand-binding domain (pTVG-AR, MVI-118) in patients with metastatic prostate cancer. Clin Cancer Res 2020, 26(19): 5162-5171.
- Shore ND, Morrow MP, McMullan T, Kraynyak KA, Sylvester A, Bhatt K, Cheung J, Boyer JD, Liu L, Sacchetta B et al: CD8+ T cells impact rising PSA in biochemically relapsed cancer patients using immunotherapy targeting tumor-associated antigens. Mol Ther 2020, 28(5): 1238-1250.
- Gulley JL, Madan RA, Tsang KY, Jochems C, Marté JL, Farsaci B, Tucker JA, Hodge JW, Liewehr DJ, Steinberg SM et al: Immune impact induced by PROSTVAC (PSA-TRICOM), a therapeutic vaccine for prostate cancer. Cancer Immunol Res 2014, 2(2): 133-141.
- Kantoff PW, Gulley JL, Pico-Navarro C: Revised overall survival analysis of a phase II, randomized, double-blind, controlled study of PROSTVAC in men with metastatic castration-resistant prostate cancer. J Clin Oncol 2017, 35(1): 124-125.
- Gulley JL, Borre M, Vogelzang NJ, Ng S, Agarwal N, Parker CC, Pook DW, Rathenborg P, Flaig TW, Carles J et al: Phase III trial of PROSTVAC in asymptomatic or minimally symptomatic metastatic castration-resistant prostate cancer. J Clin Oncol 2019, 37(13): 1051-1061.
- Stenzl A: Re: Phase III Trial of PROSTVAC in Asymptomatic or Minimally Symptomatic Metastatic Castration-resistant Prostate Cancer. Eur Urol 2019, 77(1): 131-132.
- Simons JW, Sacks N: Granulocyte-macrophage colony-stimulating factor− transduced allogeneic cancer cellular immunotherapy: The GVAX® vaccine for prostate cancer. Urol Oncol 2006, 24(5): 419-424.
- Gamat-Huber M, Jeon D, Johnson LE, Moseman JE, Muralidhar A, Potluri HK, Rastogi I, Wargowski E, Zahm CD, McNeel DG et al: Treatment combinations with DNA vaccines for the treatment of metastatic castration-resistant prostate cancer (mCRPC). Cancers 2020, 12(10): 2831.
- Obradovic AZ, Dallos MC, Zahurak ML, Partin AW, Schaeffer EM, Ross AE, Allaf ME, Nirschl TR, Liu D, Chapman CG et al: T-cell infiltration and adaptive Treg resistance in response to androgen deprivation with or without vaccination in localized prostate cancer. Clin Cancer Res 2020, 26(13): 3182-3192.
- Rosenberg SA, Restifo NP, Yang JC, Morgan RA, Dudley ME: Adoptive cell transfer: a clinical path to effective cancer immunotherapy. Nat Rev Cancer 2008, 8(4): 299-308.
- Fu J, Shang Y, Qian Z, Hou J, Yan F, Liu G, Dehua L, Tian X: Chimeric Antigen receptor-T (CAR-T) cells targeting Epithelial cell adhesion molecule (EpCAM) can inhibit tumor growth in ovarian cancer mouse model. J Vet Med Sci 2021, 83(2): 241-247.
- Bębnowska D, Grywalska E, Niedźwiedzka-Rystwej P, Sosnowska-Pasiarska B, Smok-Kalwat J, Pasiarski M, Góźdź S, Roliński J, Polkowski W: CAR-T cell therapy-an overview of targets in gastric cancer. J Clin Med 2020, 9(6): 1894.
- He C, Zhou Y, Li Z, Farooq MA, Ajmal I, Zhang H, Zhang L, Tao L, Yao J, Du B et al: Co-expression of IL-7 improves NKG2D-based CAR T cell therapy on prostate cancer by enhancing the expansion and inhibiting the apoptosis and exhaustion. Cancers 2020, 12(7): 1969.
- Narayan V, Barber-Rotenberg JS, Jung IY, Lacey SF, Rech AJ, Davis MM, Hwang WT, Lal P, Carpenter EL, Maude SL et al: PSMA-targeting TGFβ-insensitive armored CAR T cells in metastatic castration-resistant prostate cancer: a phase 1 trial. Nat Med 2022, 28(4): 724-734.
- Rosa K: OncLive https://www.onclive.com/ view/p-psma-101-elicits-encouraging-response in metastatic castrationresistant prostate cancer (May 2022).
- Krueger TE, Thorek DL, Meeker AK, Isaacs JT, Brennen WN: Tumor‐infiltrating mesenchymal stem cells: Drivers of the immunosuppressive tumor microenvironment in prostate cancer? Prostate 2019, 79(3): 320-330.
- Li W, Zhao K, Kirberger M, Liao W, Yan Y: Next generation sequencing technologies in cancer diagnostics and therapeutics: A mini review. Cell Mol Biol 2015, 61(5): 91-102.
- Yunger S, Bar El A, Zeltzer LA, Fridman E, Raviv G, Laufer M, Schachter J, Markel G, Itzhaki O, Besser MJ et al: Tumor-infiltrating lymphocytes from human prostate tumors reveal anti-tumor reactivity and potential for adoptive cell therapy. Oncoimmunology 2019, 8(12): e1672494.
- Lee HW, Nam KO, Park SJ, Kwon BS: 4‐1BB enhances CD8+ T cell expansion by regulating cell cycle progression through changes in expression of cyclins D and E and cyclin‐dependent kinase inhibitor p27kip1. Eur J Immunol 2003, 33(8): 2133-2141.
- Sartor O, De Bono J, Chi KN, Fizazi K, Herrmann K, Rahbar K, Tagawa ST, Nordquist LT, Vaishampayan N, El-Haddad G et al: Lutetium-177–PSMA-617 for metastatic castration-resistant prostate cancer. N Engl J Med 2021, 385(12): 1091-1103.
- Kantarjian H, Stein A, Gökbuget N, Fielding AK, Schuh AC, Ribera JM, Wei A, Dombret H, Foà R, Bassan R et al: Blinatumomab versus chemotherapy for advanced acute lymphoblastic leukemia. N Engl J Med 2017, 376(9): 836-847.
- Hummel HD, Kufer P, Grüllich C, Seggewiss-Bernhardt R, Deschler-Baier B, Chatterjee M, Goebeler ME, Miller K, de Santis M, Loidl W et al: Pasotuxizumab, a BiTE® immune therapy for castration-resistant prostate cancer: Phase I, dose-escalation study findings. Immunotherapy 2021, 13(2): 125-141.
- Lund ME, Howard CB, Thurecht KJ, Campbell DH, Mahler SM, Walsh BJ: A bispecific T cell engager targeting Glypican-1 redirects T cell cytolytic activity to kill prostate cancer cells. BMC Cancer 2020, 20(1): 1214.
- Yamamoto K, Trad A, Baumgart A, Hüske L, Lorenzen I, Chalaris A, Grötzinger J, Dechow T, Scheller J, Rose-John S et al: A novel bispecific single-chain antibody for ADAM17 and CD3 induces T-cell-mediated lysis of prostate cancer cells. Biochem J 2012, 445(1): 135-144.
- Lin TY, Park JA, Long A, Guo HF, Cheung NK: Novel potent anti-STEAP1 bispecific antibody to redirect T cells for cancer immunotherapy. J Immunother Cancer 2021, 9(9) : e003114.
- Gori JL, Hsu PD, Maeder ML, Shen S, Welstead GG, Bumcrot D: Delivery and specificity of CRISPR/Cas9 genome editing technologies for human gene therapy. Hum Gene Ther 2015, 26(7): 443-451.
- Chen YH, Keiser MS, Davidson BL: Viral vectors for gene transfer. Curr Protoc Mouse Biol 2018, 8(4): e58.
- Jan R: Understanding apoptosis and apoptotic pathways targeted cancer therapeutics. Adv Pharm Bull 2019, 9(2): 205-218.
- Verma AK, Mandal S, Tiwari A, Monachesi C, Catassi GN, Srivastava A, Gatti S, Lionetti E, Catassi C: Current status and perspectives on the application of CRISPR/Cas9 gene-editing system to develop a low-gluten, non-transgenic wheat variety. Foods 2021, 10(10): 2351.
- Singh V, Khurana A, Navik U, Allawadhi P, Bharani KK, Weiskirchen R: Apoptosis and pharmacological therapies for targeting thereof for cancer therapeutics. Sci 2022, 4(2): 15.
- Sharifi N, Salmaninejad A, Ferdosi S, Bajestani AN, Khaleghiyan M, Estiar MA, Jamali M, Nowroozi MR, Shakoori A: HER2 gene amplification in patients with prostate cancer: Evaluating a CISH-based method. Oncol Lett 2016, 12(6): 4651-4658.
- Rossini A, Giussani M, Ripamonti F, Aiello P, Regondi V, Balsari A, Triulzi T, Tagliabue E: Combined targeting of EGFR and HER2 against prostate cancer stem cells. Cancer Biol Ther 2020, 21(5): 463-475.
- Jiang FN, Liang YX, Wei W, Zou CY, Chen GX, Wan YP, Liu ZZ, Yang Y, Han ZD, Zhu JG, et al: Functional classification of prostate cancer-associated miRNAs through CRISPR/Cas9-mediated gene knockout. Mol Med Rep 2020, 22(5): 3777-3784.
- Desai N, Momin M, Khan T, Gharat S, Ningthoujam RS, Omri A: Metallic nanoparticles as drug delivery system for the treatment of cancer. Exper Opin Drug Deliv 2021, 18(9): 1261-1290.
- Mount Sinai School of Medicine: Gold Nanoparticles Shown to Be Safe and Effective Treatment for Prostate Cancer. 2019. Available online: https://www.sciencedaily.com/releases/2019/08/190827123513.htm (accessed on 21 July 2022).
- Sanna V, Pala N, Sechi M: Targeted therapy using nanotechnology: focus on cancer. Int J Nanomedicine 2014, 9: 467-483.
- Yao Y, Zhou Y, Liu L, Xu Y, Chen Q, Wang Y, Wu S, Deng Y, Zhang J, Shao A et al: Nanoparticle-based drug delivery in cancer therapy and its role in overcoming drug resistance. Front Mol Biosci 2020, 7: 193.
- Friedman AD, E Claypool S, Liu R: The smart targeting of nanoparticles. Curr Pharm Des 2013, 19(35): 6315-6329.
- Matowa PR, Gundidza M, Gwanzura L, Nhachi CF: A survey of ethnomedicinal plants used to treat cancer by traditional medicine practitioners in Zimbabwe. BMC Complement Med Ther 2020, 20(1): 278.
- Sanna V, Pala N, Sechi M: Targeted therapy using nanotechnology: focus on cancer. Int J Nanomedicine 2014, 9: 467-483.
- Li K, Zhan W, Chen Y, Jha RK, Chen X: Docetaxel and doxorubicin codelivery by nanocarriers for synergistic treatment of prostate cancer. Front Pharmacol 2019, 10: 1436.
- Autio KA, Dreicer R, Anderson J, Garcia JA, Alva A, Hart LL, Milowsky MI, Posadas EM, Ryan CJ, Graf RP et al: Safety and efficacy of BIND-014, a docetaxel nanoparticle targeting prostate-specific membrane antigen for patients with metastatic castration-resistant prostate cancer: a phase 2 clinical trial. JAMA Oncol 2018, 4(10): 1344-1351.
- He MH, Chen L, Zheng T, Tu Y, He Q, Fu HL, Lin JC, Zhang W, Shu G, He L et al: Potential applications of nanotechnology in urological cancer. Front Pharmacol 2018, 9: 745.
- Habib A, Jaffar G, Khalid MS, Hussain Z, Zainab SW, Ashraf Z, Haroon A, Javed R, Khalid B, Habib P: Risk factors associated with prostate cancer. J Drug Deliv Ther 2021, 11(2): 188-193.
- Strohl WR, Naso M: Bispecific T-cell redirection versus chimeric antigen receptor (CAR)-T cells as approaches to kill cancer cells. Antibodies 2019, 8(3): 41.
Annals of urologic oncology
p-ISSN: 2617-7765, e-ISSN: 2617-7773
Copyright © Ann Urol Oncol. This work is licensed under a Creative Commons Attribution-NonCommercial-No Derivatives 4.0 International (CC BY-NC-ND 4.0) License.