Review Article | Open Access
Advances in Illuminating Prostate Cancer with Emerging Phototherapies
Ali Usman1
1Department of Pharmacology, Boston University, Boston, MA 02215, USA.
Correspondence: Ali Usman (Department of Pharmacology, Boston University, Boston, MA 02215, USA; Email: usmanali@gmail.com).
Annals of Urologic Oncology 2025, 8(1): 21-29. https://doi.org/10.32948/auo.2025.01.29
Received: 25 Oct 2024 | Accepted: 19 Jan 2025 | Published online: 31 Jan 2025
Key words prostate cancer, photodynamic therapy, photothermal therapy, targeted therapy, combination therapy
PDT relies on the combination of a molecular oxygen, light and photosensitizer to generate reactive oxygen species that can induce the death of tumor cells [22]. Photosensitizer molecules capture light at specific wavelengths, which triggers a process that activates them and results in the targeted elimination of abnormal cells [23]. This approach leads to tumor destruction via three primary mechanisms: activation of an immune response, disruption of the tumor vasculature, and direct cell death [22, 24]. PDT can provoke and modulate immune responses that contribute to long-term tumor control [23]. PDT can modulate the adaptive immune response, either enhancing or inhibiting it, depending on the specific treatment protocol used [25]. The oxidative damage caused by PDT can initiate an inflammatory response, resulting in the release of pro-inflammatory mediators that attract innate immune cells. These cells, in turn, activate a systemic anti-tumor immune response. Additionally, increasing evidence indicates that PDT aids in tumor cell destruction by boosting T-cell-mediated immune responses and fostering long-lasting immune memory [26]. PDT can induce the disruption of the tumor's microvasculature, leading to impaired blood supply and subsequent tumor cell death. This effect is achieved by targeting the photosensitizers to the blood vessels and using a brief drug-light interval [23]. The vascular effects of PDT can be specifically directed to the tumor and its surrounding tissues, offering significant advantages over other PDT protocols that focus on photosensitizer accumulation within tumor cells themselves [27]. In addition to programmed apoptotic cell death, PDT also induces non-programmed necrotic cell death [28, 29], that results in the release of cellular components and molecules that provoke an inflammatory response [30]. PDT has been shown to induce several non-conventional cell death pathways in cancer cells, as well such as pyroptosis, necroptosis, ferroptosis, paraptosis and parthanatos [29]. In particular, the necrosis of tumor cells and vasculature induced by PDT can also activate CD8+ cytotoxic T lymphocytes, which target and destroy tumor cells while circulating in the body for extended periods [23, 28].
PTT operates independently of oxygen and utilizes NIR laser absorbers to generate heat, enabling thermal ablation of cancer cells under NIR laser exposure [15, 16]. Photothermal agents are administered intravenously or directly into the tumor, where they accumulate at the targeted site. When exposed to light at specific wavelengths, these agents absorb the light energy, causing them to shift from their ground state to an excited singlet state. The energy is then released through vibrational relaxation, a non-radiative decay process, in which the molecules return to their ground state by transferring energy to nearby molecules [31]. The elevation of kinetic energy during PTT leads to a rise in local tissue temperatures, thereby heating the surrounding microenvironment. When tissues reach 41°C, a heat-shock response is activated. This response leads to rapid changes in gene expression to counteract heat-induced damage, primarily through the production of heat-shock proteins [32]. At 42°C, tissue damage becomes irreversible. Prolonged exposure to temperatures between 42°C and 46°C for 10 minutes leads to substantial cell death. In the 46–52°C range, rapid cell death occurs due to microvascular thrombosis and subsequent ischemia. When tissue temperatures surpass 60°C, immediate cell death results from protein denaturation and the destruction of the plasma membrane [33]. However, research has demonstrated that temperatures surpassing 49 °C predominantly induce necrotic cell death, which may lead to inflammation and promote secondary tumor growth [34]. Notably, maintaining a highly controlled temperature range of 46–49 °C has been identified as optimal for eradicating tumors [35]. Within the NIR spectrum, the NIR-I window of 750–900 nm is currently the most widely used range in PTT research. However, there is growing interest in exploring the NIR-II range (wavelengths exceeding 1000 nm) to enhance therapeutic efficacy as the longer wavelengths of the NIR-II region reduce tissue scattering and have lower photon energy, enabling even greater tissue penetration depths [36]. Overall, phototherapies offers promising avenues in limiting tumor burden through innovative and targeted approaches.
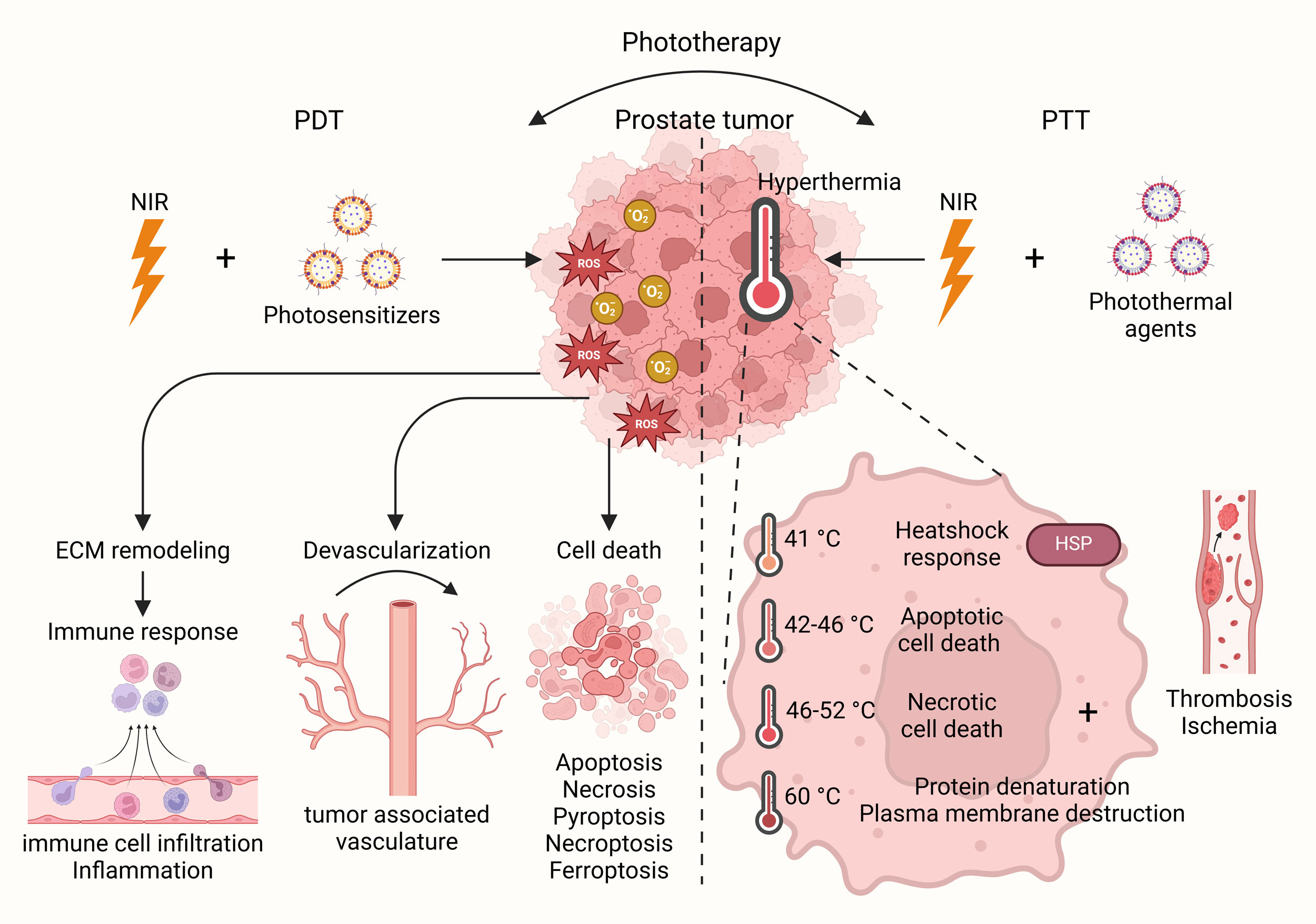
Photoimmunotherapy is an innovative treatment approach that integrates phototherapy and immunotherapy to combat cancer. This method aims to stimulate immune responses against tumors and prevent cancer recurrence [54]. This method selectively eradicates cancer cells by triggering the activating an immune response. NIR-photoimmunotherapy using the anti-prostate-specific membrane antigen (PSMA)-IR700 antibody demonstrated specific tumor targeting, significant tumor growth inhibition, and prolonged survival in a PSMA-expressing prostate cancer mouse model, with more than two-thirds of tumors cured. These findings suggest that anti-PSMA-IR700 is a promising therapeutic approach for PSMA-expressing tumors, with strong potential for clinical translation in humans [55]. Both PDT and sonodynamic therapy use sensitizers capable of producing reactive oxygen species that damage cancer cell membranes. Preclinical studies in other cancer types, such as brain cancer, have demonstrated positive outcomes from combining these two therapies [56]. While there are no direct studies investigating the combination of PDT and sonodynamic therapy for prostate cancer, ongoing research into their potential in cancer treatment is promising [57]. In the context of prostate cancer, ultrasound's deep penetration can activate photosensitizers without the need for an endoscope or light in the bladder, offering a unique advantage [56, 57]. Research is underway to identify the most effective sensitizers and delivery mechanisms to optimize the potential of PDT and its combination with other therapeutic modules in prostate cancer treatment. Overall, combining PDT with other conventional therapies is a promising avenue to reduce prostate cancer burden.
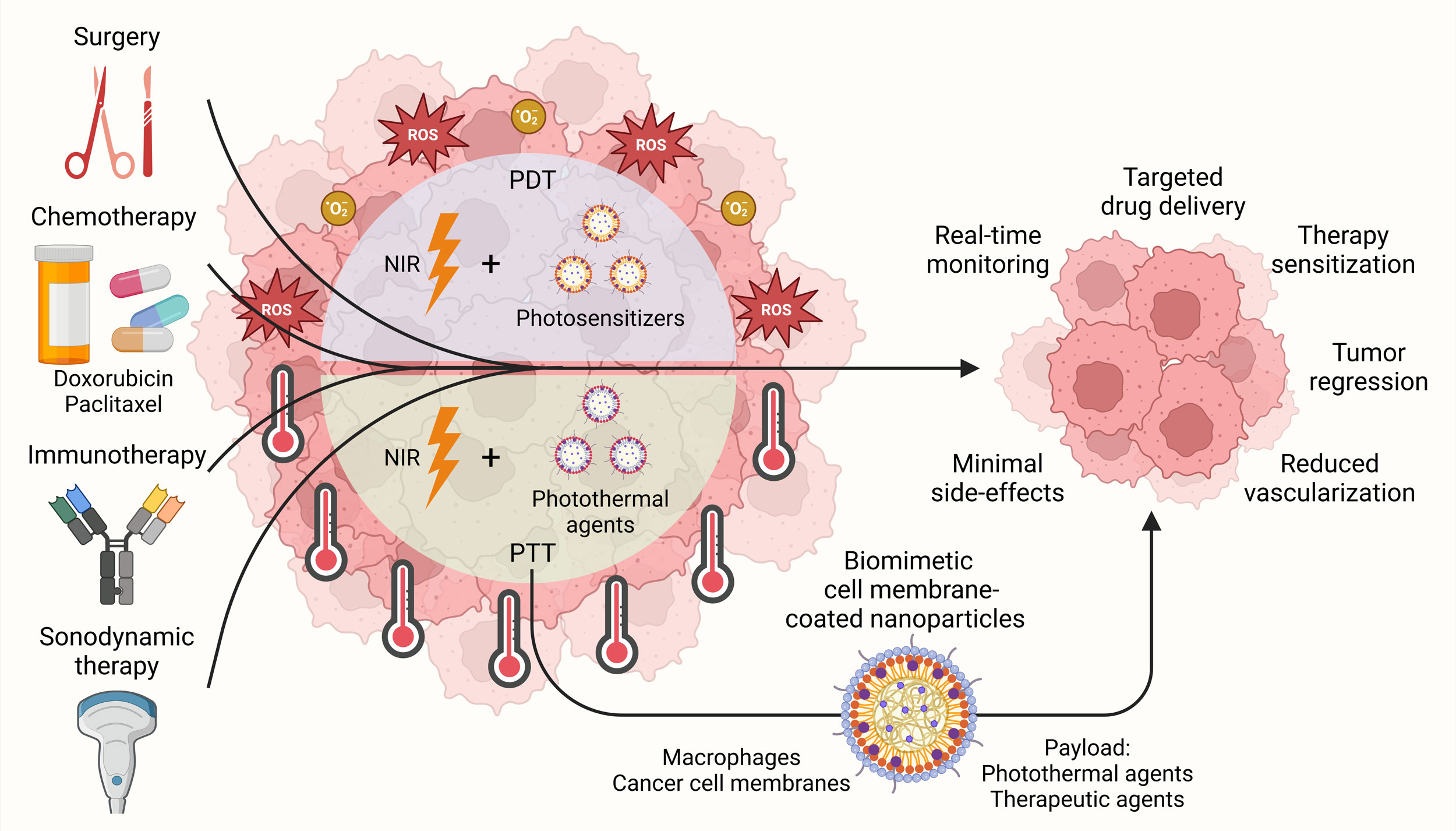
The potential of combining PTT with other therapeutic strategies for prostate cancer has garnered substantial attention in recent years as combination treatment modalities enhance overall therapeutic outcomes (Figure 2) [64]. Recent studies have explored various synergistic approaches. For instance, PSMA-targeted light-responsive nanosystems combining PTT and chemotherapy have been devised. These systems were designed with liquid perfluorocarbon cores and polymer shells, incorporating IR780 (an indocyanine green derivative) along with the chemotherapeutic agent paclitaxel.The perfluorocarbon component facilitated ultrasound-enhanced drug delivery and release via a gas–liquid transition, while IR780 enabled photoacoustic imaging-guided PTT. The combined therapy demonstrated excellent inhibitory effects on tumor cell proliferation in vitro and significant therapeutic efficacy in vivo [65]. In another approach, a Cu-MNCS-AIPH@PAA nanoplatform with excellent photothermal conversion and fenton-like reaction efficiency, have been developed. This platform synergistically combines PTT, chemodynamic therapy, and alkyl radical production, effectively inhibiting tumor growth in vitro and in vivo [66]. Similarly, SPION-based Eto delivery systems combined with mild hyperthermia (808 nm laser irradiation) to target androgen-dependent and independent prostate cancer cells. Their Eto-BSA@PAA@SPIONs showed increased drug release and cell sensitization to chemotherapy, significantly improving cytotoxicity and inducing ~50% more Eto release in nanoparticles [67]. A multifunctional nanoparticle system based on mesoporous polydopamine for targeted drug delivery and chemo-PTT of prostate cancer have been explored. The nanoparticles, AS1411@MPDA-DTX, were functionalized with the aptamer AS1411, which specifically binds to surface nucleolin expressed on prostate cancer cells. The system demonstrated effective tumor targeting, pH-responsive drug release, and enhanced anti-cancer efficacy under NIR irradiation in vitro and in vivo [68]. Additionally, doxorubicin-supported reduced graphene oxide (RGO-HNP) nanoparticles have been introduced which are stabilized by chitosan. This system achieved controlled doxorubicin release (~50% over 48 hours), high biocompatibility, and efficient heat conversion under 808 nm irradiation, demonstrating significant tumor cell death [69]. Furthermore, PLGA-ICG-R848 nanoparticles co-loaded with indocyanine green and TLR7/8 agonist R848 have been developed. Under NIR laser irradiation, this system inhibited prostate cancer cell growth and enhanced anti-tumor immunity in a mouse model, as evidenced by increased dendritic cell maturation [70]. Moreover, another platform named GCR-CDP8MA for IR-II-mediated photothermal immunotherapy has also been devised. Functionalized with gamma-Cd cross-linked polyethylenimine, targeting peptides, and meclofenamic acid, this system exhibited remarkable biocompatibility and efficient photothermal conversion when exposed to 1208 nm laser irradiation. It enhanced m6A RNA methylation, reducing PD-L1 transcript stability and promoting immune clearance through T-cell activation and maturation of dendritic cells [71]. Overall, combining PTT with other conventional therapies is a promising avenue to reduce prostate cancer burden.
Despite advances in understanding the mechanisms behind phototherapies and creating innovative nanomaterials, challenges such as lack of highly efficient photosensitizers and photothermal agents, optimizing light delivery, minimizing systemic toxicity, and scaling production for clinical use remain [38]. Multimodal therapies combining PDT with other treatments have shown potential in addressing issues related to multidrug-resistant and hypoxia-induced prostate cancers [80]. However, these strategies introduce complexities, such as increased side effects and the need for careful optimization of sequencing and timing. There has been a global effort to develop novel organic photosensitizers to improve the efficiency of PDT [81]. In recent advancements, PDT has entered a new phase involving vectorization and encapsulation techniques within drug delivery systems. These innovative strategies are aimed at improving the targeting and delivery of photosensitizers directly to tumor tissues [82-84]. Over the past five years, clinical research on the use of PTT for prostate cancer has been limited, with many studies focusing on the application of gold nanoparticles as the primary photothermal agents [85]. The limited number of clinical studies stems mainly from difficulties associated with the prostate's deep anatomical location and the prolonged process of advancing photothermal agents from basic research to clinical trials. Additionally, a significant drawback of PTT for solid tumor treatment is the inadequate penetration of light, which may prevent complete tumor eradication and increase the risk of metastasis or recurrence [86]. In addition, full potential of combining PDT and PTT is also challenged by challenges remain with the low photoconversion efficiency of some materials [87], warranting considerable improvement to enhance the efficacy of these phototherpies against prostate cancer. Lastly, further clinical studies are essential to explore the effectiveness of phototherapies, both as a standalone treatment and in combination with other cancer therapies [13]. As these therapies continue to evolve, they hold the potential to significantly improve outcomes for patients with prostate cancer, particularly those with advanced or treatment-resistant forms of the disease. By integrating phototherapies into standard care protocols, the global burden of prostate cancer could be markedly reduced, paving the way for more personalized and effective treatment strategies.
None.
Ethical policy
Non applicable.
Availability of data and materials
All data generated or analysed during this study are included in this publication.
Author contributions
AU searched academic literature, wrote the draft manuscript, drew the figures and submitted the final manuscript online.
Competing interests
None.
Funding
None.
- Bray F, Laversanne M, Sung H, Ferlay J, Siegel RL, Soerjomataram I, Jemal A: Global cancer statistics 2022: GLOBOCAN estimates of incidence and mortality worldwide for 36 cancers in 185 countries. CA Cancer J Clin 2024, 74(3): 229-263.
- Sung H, Ferlay J, Siegel RL, Laversanne M, Soerjomataram I, Jemal A, Bray F: Global Cancer Statistics 2020: GLOBOCAN Estimates of Incidence and Mortality Worldwide for 36 Cancers in 185 Countries. CA Cancer J Clin 2021, 71(3): 209-249.
- Foreman KJ, Marquez N, Dolgert A, Fukutaki K, Fullman N, McGaughey M, Pletcher MA, Smith AE, Tang K, Yuan CW, et al: Forecasting life expectancy, years of life lost, and all-cause and cause-specific mortality for 250 causes of death: reference and alternative scenarios for 2016-40 for 195 countries and territories. Lancet 2018, 392(10159): 2052-2090.
- Wang K, Ruan H, Xu T, Liu L, Liu D, Yang H, Zhang X, Chen K: Recent advances on the progressive mechanism and therapy in castration-resistant prostate cancer. Onco Targets Ther 2018, 11: 3167-3178.
- Qi Z, Xu Z, Zhang L, Zou Y, Li J, Yan W, Li C, Liu N, Wu H: Overcoming resistance to immune checkpoint therapy in PTEN-null prostate cancer by intermittent anti-PI3Kα/β/δ treatment. Nat Commun 2022, 13(1): 182.
- Wilkinson S, Ye H, Karzai F, Harmon SA, Terrigino NT, VanderWeele DJ, Bright JR, Atway R, Trostel SY, Carrabba NV, et al: Nascent Prostate Cancer Heterogeneity Drives Evolution and Resistance to Intense Hormonal Therapy. Eur Urol 2021, 80(6): 746-757.
- Wang K, Ma F, Arai S, Wang Y, Varkaris A, Poluben L, Voznesensky O, Xie F, Zhang X, Yuan X, et al: WNT5a Signaling through ROR2 Activates the Hippo Pathway to Suppress YAP1 Activity and Tumor Growth. Cancer Res 2023, 83(7): 1016-1030.
- Kishan AU, Sun Y, Hartman H, Pisansky TM, Bolla M, Neven A, Steigler A, Denham JW, Feng FY, Zapatero A, et al: Androgen deprivation therapy use and duration with definitive radiotherapy for localised prostate cancer: an individual patient data meta-analysis. Lancet Oncol 2022, 23(2): 304-316.
- Lestingi JFP, Guglielmetti GB, Trinh QD, Coelho RF, Pontes J, Jr., Bastos DA, Cordeiro MD, Sarkis AS, Faraj SF, Mitre AI, et al: Extended Versus Limited Pelvic Lymph Node Dissection During Radical Prostatectomy for Intermediate- and High-risk Prostate Cancer: Early Oncological Outcomes from a Randomized Phase 3 Trial. Eur Urol 2021, 79(5): 595-604.
- Rebello RJ, Oing C, Knudsen KE, Loeb S, Johnson DC, Reiter RE, Gillessen S, Van der Kwast T, Bristow RG: Prostate cancer. Nat Rev Dis Primers 2021, 7(1): 9.
- Kulasegaran T, Oliveira N: Metastatic Castration-Resistant Prostate Cancer: Advances in Treatment and Symptom Management. Curr Treat Options Oncol 2024, 25(7): 914-931.
- Le TK, Duong QH, Baylot V, Fargette C, Baboudjian M, Colleaux L, Taïeb D, Rocchi P: Castration-Resistant Prostate Cancer: From Uncovered Resistance Mechanisms to Current Treatments. Cancers (Basel) 2023, 15(20): 5047.
- Wahnou H, Youlyouz-Marfak I, Liagre B, Sol V, Oudghiri M, Duval RE, Limami Y: Shining a Light on Prostate Cancer: Photodynamic Therapy and Combination Approaches. Pharmaceutics 2023, 15(6): 1767.
- Dong Z, Xue K, Verma A, Shi J, Wei Z, Xia X, Wang K, Zhang X: Photothermal therapy: a novel potential treatment for prostate cancer. Biomater Sci 2024, 12(10): 2480-2503.
- Fang L, Chen Z, Dai J, Pan Y, Tu Y, Meng Q, Diao Y, Yang S, Guo W, Li L, et al: Recent Advances in Strategies to Enhance Photodynamic and Photothermal Therapy Performance of Single-Component Organic Phototherapeutic Agents. Adv Sci (Weinh) 2025, Epub ahead of print: e2409157.
- Hemmer E, Venkatachalam N, Hyodo H, Hattori A, Ebina Y, Kishimoto H, Soga K: Upconverting and NIR emitting rare earth based nanostructures for NIR-bioimaging. Nanoscale 2013, 5(23): 11339-11361.
- Vogel A, Venugopalan V: Mechanisms of pulsed laser ablation of biological tissues. Chem Rev 2003, 103(2): 577-644.
- Weissleder R: A clearer vision for in vivo imaging. Nat Biotechnol 2001, 19(4): 316-317.
- Jacques SL: Optical properties of biological tissues: a review. Phys Med Biol 2013, 58(11): R37-61.
- Stolik S, Delgado JA, Pérez A, Anasagasti L: Measurement of the penetration depths of red and near infrared light in human "ex vivo" tissues. J Photochem Photobiol B 2000, 57(2-3): 90-93.
- Tsai MF, Chang SH, Cheng FY, Shanmugam V, Cheng YS, Su CH, Yeh CS: Au nanorod design as light-absorber in the first and second biological near-infrared windows for in vivo photothermal therapy. ACS Nano 2013, 7(6): 5330-5342.
- Beşbınar Ö B, Uyar R, Yilmazer A: Omic Evaluation of Nanomaterial-Based Photodynamic Therapy of Cancer. Methods Mol Biol 2024, 2835: 277-288.
- Kwiatkowski S, Knap B, Przystupski D, Saczko J, Kędzierska E, Knap-Czop K, Kotlińska J, Michel O, Kotowski K, Kulbacka J: Photodynamic therapy - mechanisms, photosensitizers and combinations. Biomed Pharmacother 2018, 106: 1098-1107.
- Correia JH, Rodrigues JA, Pimenta S, Dong T, Yang Z: Photodynamic Therapy Review: Principles, Photosensitizers, Applications, and Future Directions. Pharmaceutics 2021, 13(9): 1332.
- Mohan R, Schellhammer PF: Treatment options for localized prostate cancer. Am Fam Physician 2011, 84(4): 413-420.
- Rodrigues MC, de Sousa Júnior WT, Mundim T, Vale CLC, de Oliveira JV, Ganassin R, Pacheco TJA, Vasconcelos Morais JA, Longo JPF, Azevedo RB, et al: Induction of Immunogenic Cell Death by Photodynamic Therapy Mediated by Aluminum-Phthalocyanine in Nanoemulsion. Pharmaceutics 2022, 14(1): 196.
- Plekhova N, Shevchenko O, Korshunova O, Stepanyugina A, Tananaev I, Apanasevich V: Development of Novel Tetrapyrrole Structure Photosensitizers for Cancer Photodynamic Therapy. Bioengineering (Basel) 2022, 9(2): 82.
- Tan L, Shen X, He Z, Lu Y: The Role of Photodynamic Therapy in Triggering Cell Death and Facilitating Antitumor Immunology. Front Oncol 2022, 12: 863107.
- Mishchenko T, Balalaeva I, Gorokhova A, Vedunova M, Krysko DV: Which cell death modality wins the contest for photodynamic therapy of cancer? Cell Death Dis 2022, 13(5): 455.
- Hamblin MR: Photodynamic Therapy for Cancer: What's Past is Prologue. Photochem Photobiol 2020, 96(3): 506-516.
- Li C, Cheng Y, Li D, An Q, Zhang W, Zhang Y, Fu Y: Antitumor Applications of Photothermal Agents and Photothermal Synergistic Therapies. Int J Mol Sci 2022, 23(14): 7909.
- Richter K, Haslbeck M, Buchner J: The heat shock response: life on the verge of death. Mol Cell 2010, 40(2): 253-266.
- Knavel EM, Brace CL: Tumor ablation: common modalities and general practices. Tech Vasc Interv Radiol 2013, 16(4): 192-200.
- Golstein P, Kroemer G: Cell death by necrosis: towards a molecular definition. Trends Biochem Sci 2007, 32(1): 37-43.
- Christofferson DE, Yuan J: Necroptosis as an alternative form of programmed cell death. Curr Opin Cell Biol 2010, 22(2): 263-268.
- Upputuri PK, Pramanik M: Photoacoustic imaging in the second near-infrared window: a review. J Biomed Opt 2019, 24(4): 1-20.
- van Straten D, Mashayekhi V, de Bruijn HS, Oliveira S, Robinson DJ: Oncologic Photodynamic Therapy: Basic Principles, Current Clinical Status and Future Directions. Cancers (Basel) 2017, 9(2): 19.
- Zhang Y, Zhang Y, Zhang G, Wu J, Wang L, Dong Z, Zheng Y, Huang Q, Zou M, Liao R: Recent advances and clinical challenges of phototherapeutic nanoparticles in cancer monotherapy or combination therapy. Coord Chem Rev 2024, 518: 216069.
- Moore CM, Pendse D, Emberton M: Photodynamic therapy for prostate cancer--a review of current status and future promise. Nat Clin Pract Urol 2009, 6(1): 18-30.
- Li J, Wang Q, Xia G, Adilijiang N, Li Y, Hou Z, Fan Z, Li J: Recent Advances in Targeted Drug Delivery Strategy for Enhancing Oncotherapy. Pharmaceutics 2023, 15(9): 2233.
- Wang F, Li Z, Feng X, Yang D, Lin M: Advances in PSMA-targeted therapy for prostate cancer. Prostate Cancer Prostatic Dis 2022, 25(1): 11-26.
- Ding X, Bai S, Liu F, Michał N, Roman S, Peng N, Liu Y: NIR-II-triggered photothermal therapy with Au@PDA/PEG-PI for targeted downregulation of PSMA in prostate cancer. Acta Biomater 2023, 157: 487-499.
- Xia L, Meng X, Wen L, Zhou N, Liu T, Xu X, Wang F, Cheng Z, Yang Z, Zhu H: A Highly Specific Multiple Enhancement Theranostic Nanoprobe for PET/MRI/PAI Image-Guided Radioisotope Combined Photothermal Therapy in Prostate Cancer. Small 2021, 17(21): e2100378.
- Algorri JF, Ochoa M, Roldán-Varona P, Rodríguez-Cobo L, López-Higuera JM: Photodynamic Therapy: A Compendium of Latest Reviews. Cancers (Basel) 2021, 13(17): 4447.
- Xue Q, Zhang J, Jiao J, Qin W, Yang X: Photodynamic therapy for prostate cancer: Recent advances, challenges and opportunities. Front Oncol 2022, 12: 980239.
- Ito Y, Udo K, Vertosick EA, Sjoberg DD, Vickers AJ, Al-Ahmadie HA, Chen YB, Gopalan A, Sirintrapun SJ, Tickoo SK, et al: Clinical Usefulness of Prostate and Tumor Volume Related Parameters following Radical Prostatectomy for Localized Prostate Cancer. J Urol 2019, 201(3): 535-540.
- Osuchowski M, Aebisher D, Bartusik-Aebisher D, Krupka-Olek M, Dynarowicz K, Przygoda M, Kawczyk-Krupka A: Photodynamic Therapy-Adjunctive Therapy in the Treatment of Prostate Cancer. Diagnostics (Basel) 2022, 12(5): 1113.
- Borgia F, Giuffrida R, Caradonna E, Vaccaro M, Guarneri F, Cannavò SP: Early and Late Onset Side Effects of Photodynamic Therapy. Biomedicines 2018, 6(1): 12.
- Lebdai S, Villers A, Barret E, Nedelcu C, Bigot P, Azzouzi AR: Feasibility, safety, and efficacy of salvage radical prostatectomy after Tookad® Soluble focal treatment for localized prostate cancer. World J Urol 2015, 33(7): 965-971.
- Pierrard V, Lebdai S, Kleinclauss F, Azzouzi AR, Terrier JE, Fortier E, Joniau S, Van Der Poel H, Salomon G, Casanova J, et al: Radical Prostatectomy after Vascular Targeted Photodynamic Therapy with Padeliporfin: Feasibility, and Early and Intermediate Results. J Urol 2019, 201(2): 315-321.
- Li G, Wang C, Jin B, Sun T, Sun K, Wang S, Fan Z: Advances in smart nanotechnology-supported photodynamic therapy for cancer. Cell Death Discov 2024, 10(1): 466.
- Poudel BK, Soe ZC, Ruttala HB, Gupta B, Ramasamy T, Thapa RK, Gautam M, Ou W, Nguyen HT, Jeong JH, et al: In situ fabrication of mesoporous silica-coated silver-gold hollow nanoshell for remotely controllable chemo-photothermal therapy via phase-change molecule as gatekeepers. Int J Pharm 2018, 548(1): 92-103.
- Wang X, Tong J, He Z, Yang X, Meng F, Liang H, Zhang X, Luo L: Paclitaxel-Potentiated Photodynamic Theranostics for Synergistic Tumor Ablation and Precise Anticancer Efficacy Monitoring. ACS Appl Mater Interfaces 2020, 12(5): 5476-5487.
- Mohiuddin TM, Zhang C, Sheng W, Al-Rawe M, Zeppernick F, Meinhold-Heerlein I, Hussain AF: Near Infrared Photoimmunotherapy: A Review of Recent Progress and Their Target Molecules for Cancer Therapy. Int J Mol Sci 2023, 24(3): 2655.
- Nagaya T, Nakamura Y, Okuyama S, Ogata F, Maruoka Y, Choyke PL, Kobayashi H: Near-Infrared Photoimmunotherapy Targeting Prostate Cancer with Prostate-Specific Membrane Antigen (PSMA) Antibody. Mol Cancer Res 2017, 15(9): 1153-1162.
- Borah BM, Cacaccio J, Durrani FA, Bshara W, Turowski SG, Spernyak JA, Pandey RK: Sonodynamic therapy in combination with photodynamic therapy shows enhanced long-term cure of brain tumor. Sci Rep 2020, 10(1): 21791.
- Hu X, Zhang YS, Liu YC, Wang N, Zeng XT, Zhang LL: Emerging photodynamic/sonodynamic therapies for urological cancers: progress and challenges. J Nanobiotechnology 2022, 20(1): 437.
- Du B, Ma C, Ding G, Han X, Li D, Wang E, Wang J: Cooperative Strategies for Enhancing Performance of Photothermal Therapy (PTT) Agent: Optimizing Its Photothermal Conversion and Cell Internalization Ability. Small 2017, 13(13): 1603275.
- Li S, Ma Y, Ma C, Shi L, Li F, Chang L: NIR-triggerable self-assembly multifunctional nanocarriers to enhance the tumor penetration and photothermal therapy efficiency for castration-resistant prostate cancer. Discov Nano 2023, 18(1): 46.
- Kim J, Chun SH, Amornkitbamrung L, Song C, Yuk JS, Ahn SY, Kim BW, Lim YT, Oh BK, Um SH: Gold nanoparticle clusters for the investigation of therapeutic efficiency against prostate cancer under near-infrared irradiation. Nano Converg 2020, 7(1): 5.
- Zheng Z, Yu P, Cao H, Cheng M, Zhou T, Lee LE, Ulstrup J, Zhang J, Engelbrekt C, Ma L: Starch Capped Atomically Thin CuS Nanocrystals for Efficient Photothermal Therapy. Small 2021, 17(47): e2103461.
- Wang Z, He L, Che S, Xing H, Guan L, Yang Z, Li X, Zvyagin AV, Lin Q, Qu W: AuNCs-LHRHa nano-system for FL/CT dual-mode imaging and photothermal therapy of targeted prostate cancer. J Mater Chem B 2022, 10(27): 5182-5190.
- Wang Z, Xing H, Liu A, Guan L, Li X, He L, Sun Y, Zvyagin AV, Yang B, Lin Q: Multifunctional nano-system for multi-mode targeted imaging and enhanced photothermal therapy of metastatic prostate cancer. Acta Biomater 2023, 166: 581-592.
- Ferroni C, Del Rio A, Martini C, Manoni E, Varchi G: Light-Induced Therapies for Prostate Cancer Treatment. Front Chem 2019, 7: 719.
- Xiao L, Wu Y, Dai J, Zhang W, Cao Y: Laser-activated nanoparticles for ultrasound/photoacoustic imaging-guided prostate cancer treatment. Front Bioeng Biotechnol 2023, 11: 1141984.
- Xu B, Niu R, Tang Y, Wang C, Jin L, Wang Y: A Cu-based nanoplatform for near-infrared light amplified multi-mode prostate cancer specific therapy. J Mater Chem B 2022, 10(37): 7628-7633.
- Onbasli K, Erkısa M, Demirci G, Muti A, Ulukaya E, Sennaroglu A, Yagci Acar H: The improved killing of both androgen-dependent and independent prostate cancer cells by etoposide loaded SPIONs coupled with NIR irradiation. Biomater Sci 2022, 10(14): 3951-3962.
- Dai L, Wei D, Zhang J, Shen T, Zhao Y, Liang J, Ma W, Zhang L, Liu Q, Zheng Y: Aptamer-conjugated mesoporous polydopamine for docetaxel targeted delivery and synergistic photothermal therapy of prostate cancer. Cell Prolif 2021, 54(11): e13130.
- SreeHarsha N, Maheshwari R, Al-Dhubiab BE, Tekade M, Sharma MC, Venugopala KN, Tekade RK, Alzahrani AM: Graphene-based hybrid nanoparticle of doxorubicin for cancer chemotherapy. Int J Nanomedicine 2019, 14: 7419-7429.
- Lin W, Li C, Xu N, Watanabe M, Xue R, Xu A, Araki M, Sun R, Liu C, Nasu Y, et al: Dual-Functional PLGA Nanoparticles Co-Loaded with Indocyanine Green and Resiquimod for Prostate Cancer Treatment. Int J Nanomedicine 2021, 16: 2775-2787.
- Liu J, Song Y, Wang Y, Han M, Wang C, Yan F: Cyclodextrin-Functionalized Gold Nanorods Loaded with Meclofenamic Acid for Improving N(6)-Methyladenosine-Mediated Second Near-Infrared Photothermal Immunotherapy. ACS Appl Mater Interfaces 2022, 14(36): 40612-40623.
- Huang X, Chen L, Lin Y, Tou KI, Cai H, Jin H, Lin W, Zhang J, Cai J, Zhou H, et al: Tumor targeting and penetrating biomimetic mesoporous polydopamine nanoparticles facilitate photothermal killing and autophagy blocking for synergistic tumor ablation. Acta Biomater 2021, 136: 456-472.
- Qiang L, Cai Z, Jiang W, Liu J, Tai Z, Li G, Gong C, Gao S, Gao Y: A novel macrophage-mediated biomimetic delivery system with NIR-triggered release for prostate cancer therapy. J Nanobiotechnology 2019, 17(1): 83.
- Liu L, Yang S, Zheng Z, Li Q, Liu C, Hu D, Liu Z, Zhang X, Zhang R, Gao D: Biomimetic Theranostic Agents with Superior NIR-II Photoacoustic and Magnetic Resonance Imaging Performance for Targeted Photothermal Therapy of Prostate Cancer. Pharmaceutics 2023, 15(6): 1617.
- Huang L, Xu C, Xu P, Qin Y, Chen M, Feng Q, Pan J, Cheng Q, Liang F, Wen X, et al: Intelligent Photosensitive Mesenchymal Stem Cells and Cell-Derived Microvesicles for Photothermal Therapy of Prostate Cancer. Nanotheranostics 2019, 3(1): 41-53.
- Thapa RK, Soe ZC, Ou W, Poudel K, Jeong JH, Jin SG, Ku SK, Choi HG, Lee YM, Yong CS, et al: Palladium nanoparticle-decorated 2-D graphene oxide for effective photodynamic and photothermal therapy of prostate solid tumors. Colloids Surf B Biointerfaces 2018, 169: 429-437.
- Wang Q, Zhang X, Sun Y, Wang L, Ding L, Zhu WH, Di W, Duan YR: Gold-caged copolymer nanoparticles as multimodal synergistic photodynamic/photothermal/chemotherapy platform against lethality androgen-resistant prostate cancer. Biomaterials 2019, 212: 73-86.
- Tan H, Hou N, Liu Y, Liu B, Cao W, Zheng D, Li W, Liu Y, Xu B, Wang Z, et al: CD133 antibody targeted delivery of gold nanostars loading IR820 and docetaxel for multimodal imaging and near-infrared photodynamic/photothermal/chemotherapy against castration resistant prostate cancer. Nanomedicine 2020, 27: 102192.
- Hu J, Luo H, Qu Q, Liao X, Huang C, Chen J, Cai Z, Bao Y, Chen G, Li B, et al: Cell Membrane-Inspired Polymeric Vesicles for Combined Photothermal and Photodynamic Prostate Cancer Therapy. ACS Appl Mater Interfaces 2020, 12(38): 42511-42520.
- Sarbadhikary P, George BP, Abrahamse H: Recent Advances in Photosensitizers as Multifunctional Theranostic Agents for Imaging-Guided Photodynamic Therapy of Cancer. Theranostics 2021, 11(18): 9054-9088.
- Pham TC, Nguyen VN, Choi Y, Lee S, Yoon J: Recent Strategies to Develop Innovative Photosensitizers for Enhanced Photodynamic Therapy. Chem Rev 2021, 121(21): 13454-13619.
- Gallardo-Villagrán M, Paulus L, Charissoux JL, Sutour S, Vergne-Salle P, Leger DY, Liagre B, Therrien B: Evaluation of Ruthenium-Based Assemblies as Carriers of Photosensitizers to Treat Rheumatoid Arthritis by Photodynamic Therapy. Pharmaceutics 2021, 13(12): 2104.
- Nkune NW, Matlou GG, Abrahamse H: Photodynamic Therapy Efficacy of Novel Zinc Phthalocyanine Tetra Sodium 2-Mercaptoacetate Combined with Cannabidiol on Metastatic Melanoma. Pharmaceutics 2022, 14(11): 2418.
- Dandash F, Leger DY, Diab-Assaf M, Sol V, Liagre B: Porphyrin/Chlorin Derivatives as Promising Molecules for Therapy of Colorectal Cancer. Molecules 2021, 26(23): 7268.
- Riley RS, Day ES: Gold nanoparticle-mediated photothermal therapy: applications and opportunities for multimodal cancer treatment. Wiley Interdiscip Rev Nanomed Nanobiotechnol 2017, 9(4): 10.
- Pinho S, Coelho JMP, Gaspar MM, Reis CP: Advances in localized prostate cancer: A special focus on photothermal therapy. Eur J Pharmacol 2024, 983: 176982.
- Overchuk M, Weersink RA, Wilson BC, Zheng G: Photodynamic and Photothermal Therapies: Synergy Opportunities for Nanomedicine. ACS Nano 2023, 17(9): 7979-8003.
Annals of urologic oncology
p-ISSN: 2617-7765, e-ISSN: 2617-7773
Copyright © Ann Urol Oncol. This work is licensed under a Creative Commons Attribution-NonCommercial-No Derivatives 4.0 International (CC BY-NC-ND 4.0) License.